Clinical use
General assay characteristics
Specific clinical applications
Diagnosis
Rapid turnaround time thereby facilitating targeted antiviral therapy
Highly specific for the pathogen of interest
More sensitive than conventional methods such as culture
High sensitivity may detect latent virus: viral quantification or detection of viral RNA may aid in the differentiation of replicating from latent virus
Nucleic acid tests for adenovirus, BK virus, parvovirus B19, herpes simplex virus, varicella zoster virus, cytomegalovirus, Epstein Barr virus, and other herpesviruses are sensitive and rapid methods for confirming clinical suspicion of specific viral diseases
Surveillance
Guide initiation of preemptive therapy strategy
Identifies patients at high risk of clinical disease (risk stratification)
Identifies patients at high risk of treatment failure and relapse (prognosis)
Surveillance for cytomegalovirus infection by nucleic acid testing guides the initiation of antiviral treatment prior to the onset of clinical disease
Surveillance for BK virus in kidney transplant recipients identifies infection prior to the onset of nephropathy, thereby allowing preemptive reduction in immunosuppression as treatment
Surveillance for Epstein Barr virus infection identifies patients at high risk of post-transplant lymphoproliferative disease
High cytomegalovirus and Epstein Barr virus viral loads indicate high risk of clinical disease, although widely applicable viral thresholds have been difficult to define due to lack of assay standardization
Monitor treatment responses
Guide the duration of antiviral therapy
Early indicator of risk of treatment failure
Early indicator of disease relapse
Early indicator of the emergence of resistant viruses
Treatment of cytomegalovirus disease is generally continued until the virus is undetectable by a sensitive PCR test
Viral load testing for cytomegalovirus, Epstein Barr virus, and BK virus generally decline following initiation of a successful treatment strategy
Rise in cytomegalovirus, Epstein Barr virus, and BK virus viral load during treatment indicates relapse, an over-immunosuppressed state, or the possible emergence of drug resistance
The increased sensitivity of molecular tests for viral detection has posed an important challenge in differentiating active from latent infection. A number of viruses that cause clinical disease in transplant recipients exist in a latent state, and there is concern that highly sensitive molecular assays may detect nucleic acid from such latent, non-replicating viruses in the absence of active disease. If not interpreted in the proper clinical context, this may lead to unnecessary treatment.
The majority of molecular tests for viral diagnosis in transplantation lack standardization, and their clinical use across different testing sites has been difficult, if not impossible [3, 4]. Most viral laboratory-developed tests (LDTs) do not use international standards, and hence, the performance characteristics vary widely and the results are not directly comparable. Variability in specimen type (plasma, whole blood, or peripheral blood leukocytes), nucleic acid extraction methods, target sequence, limit of detection, and quantitative standard are just some of the reasons why values obtained from different LDTs are not directly comparable (Table 47.2). This lack of standardization not only complicates management of individual patients, but also hampers the development of consensus interpretive and treatment guidelines.
Table 47.2
Factors contributing to the variability in viral load reporting among different laboratories
Assay platform |
Clinical specimen type |
Pipetting technique |
Nucleic acid extraction method |
Molecular amplification target gene |
Probes |
Detection method |
Frequency of assay calibration |
Quantitative calibrator |
Number of amplification cycles for PCR-based methods |
This chapter discusses the clinical application of molecular tests for the diagnosis of viral infections in transplant recipients. Although many viruses can infect transplant recipients, this chapter will focus on more commonly encountered pathogens, including adenovirus, BK virus (BKV), parvovirus B19, cytomegalovirus (CMV), Epstein Barr virus (EBV), and the other members of the herpes virus family.
Adenovirus
Description of Pathogen and Clinical Utility of Testing
Adenoviruses are a family of 57 immunologically distinct, non-enveloped, double-stranded DNA viruses that cause a variety of clinical syndromes in humans [5, 6]. Each adenovirus serotype, termed groups A through G, is associated with a distinct clinical illness, which in immunocompetent hosts can manifest as self-limited respiratory, gastrointestinal, or conjunctival disease [5]. Infection with adenovirus occurs throughout the year, although a predominance of cases is observed during fall and winter months [7]. Adenovirus infections occur most commonly in children, those living in close quarters, and immunocompromised patients including transplant recipients [5].
The incidence of adenovirus infection after transplantation is estimated at 5–15 % of patients [8, 9]. In a large prospective study of 263 adult SOT recipients, adenovirus DNA was detected in the blood of 7.2 % of patients during the first year after transplantation [8]. However, this study may have underestimated the true incidence of adenoviral disease since it did not capture localized and non-viremic infections. Children have a higher incidence of adenoviral infection compared to adult patients. Adenovirus was detected in 10 % of 484 pediatric liver recipients [10], compared to only 5.8 % of 191 adult liver recipients [11]. In a cohort of pediatric allogeneic HSCT recipients, the probability of developing adenovirus infection was 12.3 % [12], while the incidence was 2.5 % in adult allogeneic HSCT recipients [13].
Adenovirus infection after transplantation may or may not be associated with clinical symptoms [5]. The clinical manifestations, rate, and severity of infection may vary based on immunosuppression level, the type of SOT or HSCT, and the time to onset since transplantation [5]. Since immunosuppression is most intense during the early period after transplantation, clinical disease is most often seen during the first 3 months after transplantation [5]. In a prospective study, 60 % of SOT patients with adenovirus DNA detectable in blood after transplantation had no clinical symptoms [8]. For the remaining 40 % of SOT patients, symptoms were related to gastrointestinal and respiratory tract infections [8]. Nonspecific symptom of fever also was observed in SOT recipients. In liver transplant recipients, infection with adenovirus serotype 5 was associated with hepatitis while serotypes 1 and 2 were associated with pneumonia [10, 11]. Adenovirus may involve the allograft, which can manifest as allograft dysfunction and may be mistaken for rejection. Adenoviral pneumonia may contribute to bronchiolitis obliterans, graft loss, or even death in lung transplant patients [14]. Adenovirus infection can cause hemorrhagic cystitis and graft dysfunction after kidney transplantation [15]. In highly immunosuppressed patients, such as allogeneic stem cell transplant recipients, adenovirus infection may manifest as disseminated disease with involvement of the liver, gastrointestinal tract, and lungs [12]. Adenovirus infection is reported as one of the most significant risk factors for death in allogeneic HSCT recipients [12].
Available Assays and Interpretation of Results
The gold standard for the diagnosis of invasive adenoviral disease is histological evaluation of a tissue specimen [5], which demonstrates typical cytopathic inclusions known as “smudge cells.” The presence of adenovirus within the tissue sample may be confirmed through the use of immunohistochemistry or in situ hybridization. Less invasive approaches to diagnosis are (1) antigen detection, (2) culture, and (3) molecular assays using blood and other fluids from affected organs. A single diagnostic test may not be able to detect all adenovirus serotypes. Enzyme immunoassays (EIAs) are typically the basis of rapid antigen detection kits. Rapid antigen detection kits are commercially available but their performance characteristics in the transplant population have not been rigorously studied. Moreover, while most EIAs can detect serotypes associated with ocular or gastrointestinal disease, they are not able to detect all adenovirus types and some have been associated with a low sensitivity of detection [5]. Rapid detection of adenovirus also can be done by immunofluorescence, using either polyclonal or monoclonal antibodies [5]. Culture techniques suffer from prolonged turnaround time. Although time to detection has improved with the introduction of the shell vial assay [5], sensitivity remains an issue.
PCR Tests for the Detection of Adenovirus
Nucleic acid detection using PCR has emerged as the preferred diagnostic tool for identification of adenoviral infections in transplant recipients [16]. Adenovirus PCR testing is generally more sensitive than culture-based methods [17]. In a study of HSCT recipients, adenovirus NAT had a higher sensitivity compared to viral culture and antigen detection [18, 19]. Numerous molecular assays are available, including commercial reagents and LDTs, with widely variable performance characteristics. The assay performance differences are (at least in part) dependent on the specimen type, primers used, and extraction method, among other factors. Whole blood, plasma, stool, urine, respiratory secretions (e.g., bronchoalveolar lavage), and other body fluids have been used. Comparability of tests is complicated by the fact that very few are cleared by the US Federal Drug Administration (FDA) for clinical in vitro diagnostic use. Some qualitative assays are US FDA cleared, but only for use with respiratory samples. Compounding the problem is the lack of internationally standardized quantitative calibrators. Many NATs will not detect all adenovirus serotypes, although a few have been developed to detect all known adenovirus serotypes [17, 20]. Some multiplex PCR systems include adenovirus in a test for simultaneous detection of many respiratory viruses [21]. However, multiplexed PCR can reduce the sensitivity of viral detection. Some of the broadly multiplexed viral tests have diminished sensitivity for adenovirus compared to adenovirus-specific tests. The large number of adenovirus serotypes and their genetic heterogeneity can complicate adequate validation of these assays. Hence, verification of NAT assay performance should include a wide variety of adenovirus serotypes, with assessment of both sensitivity and quantitative accuracy.
Quantitative Adenovirus Tests
Quantitative NAT for adenovirus can be used for risk stratification and prognostication [16, 18, 19]. Serial surveillance of the blood for adenovirus DNA in transplant recipients may identify the infection prior to the onset of clinical illness. Detection of adenoviral DNA in asymptomatic patients can allow for preemptive therapy for patients with a high risk of progression to clinical disease, such as those with high viral load, severe lymphopenia, and receiving intense immunosuppression for GVHD or allograft rejection [16]. Recent guidelines have recommended serial adenovirus surveillance of blood of high-risk allogeneic HSCT recipients to identify and predict the onset of invasive adenoviral disease [9, 17]; however, the clinical utility of this approach remains uncertain [22]. In one prospective study of 97 allogeneic HSCT recipients, surveillance of the blood identified adenovirus in five patients (5 % incidence, including one with a very high viral load), although this did not have an impact on the clinical outcome of these patients [23].
Some groups have even demonstrated the clinical utility of quantitative adenovirus PCR surveillance of stool samples to predict subsequent adenovirus viremia [16, 20]. In these studies, the detection of high levels of adenoviral DNA in the stool preceded the onset of viremia by at least 11 days [16, 18, 20]. The incidence of viremia was 73 % for those with >1 × 106 copies of adenovirus DNA per gram of stool, while patients with lower values did not develop adenoviral viremia [20]. Serial stool surveillance predicted invasive adenoviral disease with 100 % sensitivity and 83 % specificity [20].
Adenovirus may be shed asymptomatically for prolonged periods of time, and thus, recovery of adenovirus from urine, upper respiratory secretions, or stool sites by culture does not necessarily confirm adenovirus as the cause of a specific disease [24, 25]. Indeed, the detection of adenovirus in one site, such as the urine alone, has poor predictive value and, therefore is not recommended for routine surveillance. However, the detection of adenovirus at two or more sites is highly predictive of invasive disease [24, 25]. Quantitation of viral load may help differentiate true infection from subclinical shedding, although the viral load threshold for discriminating a clinically relevant infection is not defined due to a lack of studies using standardized assays.
Quantitative adenovirus NAT can be used for monitoring treatment response. Treatment of established adenovirus disease consists of cautious reduction of immunosuppression and supportive care. There is no US FDA-approved antiviral drug for the treatment of established adenoviral disease, although cidofovir (CDV) has been used most frequently [5]. Treatment responses may be monitored by quantitative adenovirus PCR. Failure of the viral load to decline by 1 log within 2 weeks of treatment, or a persistent rise in viral load has been associated with mortality [26]. Generally, a higher adenoviral DNA level in the blood is associated with poor prognosis and a higher rate of treatment failure [6]. In a study of 27 adult allogeneic HSCT recipients, the peak adenovirus DNA load was significantly higher among seven patients who died of adenovirus disease compared to those without adenovirus-related death (1 × 108 vs 3 × 105; p < 0.001) [6].
BK Virus
Description of Pathogen and Clinical Utility of Testing
BKV is a non-enveloped, double-stranded DNA virus that belongs to the family Polyomaviridae, together with JC virus (JCV) [27]. Both BKV and JCV are named by the initials of the patients from which the viruses were originally identified. Primary BKV infection is common during childhood and is typically asymptomatic. More than 90 % of adults have serologic evidence of past BKV infection. BKV is transmitted through oral or respiratory routes. After primary infection, BKV remains latent in urothelial cells and leukocytes [27]. Reactivation of latent virus resulting in asymptomatic urinary shedding may occur in up to 10 % of healthy individuals [27].
In transplant recipients, BKV reactivation is more common and may lead to cytopathic changes in the urothelium. BKV viruria has been detected in up to 40 % of kidney transplant recipients and up to 75 % of allogeneic HSCT recipients [27]. The vast majority of BKV viruria in transplant recipients is subclinical, but kidney transplant and HSCT patients with viruria at very high levels may have clinical manifestations [28–31]. High levels of urinary tract BKV replication may result in sloughing of altered epithelial cells with nuclear inclusions (known as “decoy cells”), which are excreted in the urine and may be detected by urine cytologic examination [32, 33]. Inflammation elicited by necrosis and denudation of the tubular basement membrane is followed by infiltration of lymphocytes, tubular atrophy, and fibrosis. In the absence of therapeutic intervention, approximately 30–50 % of kidney transplant patients with high-level BKV viruria progress to viremia and end organ disease.
The two major clinical syndromes associated with BKV after transplantation are BKV-associated nephropathy (BKAN; in kidney transplant recipients) [29, 34, 35] and hemorrhagic cystitis (primarily in allogeneic HSCT recipients) [28, 36]. BKAN occurs in 1–10 % of kidney transplant recipients, while recipients of other solid organ allografts rarely develop BKAN. BKAN is most commonly manifested by a rise in serum creatinine with corresponding renal histopathologic findings of varying severity, including inflammation, tubular atrophy, and fibrosis, together with viral inclusions that can be identified by electron microscopy, immunohistochemistry, or in situ hybridization. Hemorrhagic cystitis, often of late onset, affects 5–15 % of allogeneic HSCT patients [37]. Occurring in the post-engraftment period, hemorrhagic cystitis may be characterized by abdominal pain, dysuria, frequent micturition, and microscopic or gross hematuria [28, 37]. Severe hematuria leading to clot formation, ureteral obstruction, and renal failure has been reported. The other diseases associated with BKV infection are ureteric stenosis, pneumonitis, and hemophagocytic syndrome [27].
Antiviral drug treatment is not established for BKAN or for hemorrhagic cystitis [27]. Cidofovir has been used anecdotally for the treatment of BKV infection in transplant patients [27], although data to convincingly support this antiviral therapy in transplant patients is not available. A randomized controlled clinical trial comparing CDV to placebo for the treatment of BKAN has been halted due to poor enrollment of study subjects. In the absence of the proven effectiveness of antiviral therapy, reduction in immunosuppressive therapy is regarded as the first line of treatment [27], since the pathogenesis of BKAN involves an imbalance between BKV replication and BKV-specific immune control.
Available Assays and Interpretation of Results
Histopathology for BKV
Histopathology is the gold standard for diagnosis of BKAN [38]. Early and accurate diagnosis of BKAN is essential to prevent graft failure. However, often no clear clinical symptoms of BKV infection are present, and the only clinical sign may be a gradual rise in serum creatinine. Histopathology of allograft biopsy specimens with BKAN shows extensive viral cytopathic effect, necrosis of the cells of the tubules and collecting ducts, and varying degrees of interstitial inflammation [38]. The pathology is focal and patchy, and, thus, this invasive procedure may yield false-negative results due to sampling error [32]. Likewise, in HSCT patients with late onset hemorrhagic cystitis, a biopsy of the bladder epithelium is needed to demonstrate the potential role of BKV infection [37].
A noninvasive alternative for diagnosis of BKV infection is to examine the urine for the presence of decoy cells, which contain characteristic intracellular viral inclusion bodies [39]. Identification of decoy cells confirms active BKV replication, but this finding is not highly specific for BKAN or hemorrhagic cystitis. Several studies have demonstrated that the sensitivity of urinary decoy cell detection in kidney transplant recipients with BKAN is modest (and comparatively less than molecular methods). In one study, urine decoy cell detection had 25 % sensitivity and 84 % specificity in identifying four patients with BKAN among a cohort of 114 kidney transplant patients [39]; the corresponding positive predictive value for concurrent BKAN was only 5 % and the negative predictive value was 97 % [39]. Decoy cell detection has been used to support the diagnosis of late-onset hemorrhagic cystitis due to BKV in HSCT recipients, but this is nonspecific, since decoy cells can be detected in other viral infections, such as adenovirus, and may be confused with malignancy [37].
Molecular Tests for BKV
Molecular tests are the most common method for diagnosis of BKV infection in kidney transplant recipients. Diagnosis of active BKV infection is most commonly achieved using quantitative PCR (qPCR) for BKV DNA, which has been demonstrated to have better predictive value than urinary decoy cell detection for the diagnosis of BKAN. BKV qPCR can be performed using urine or blood samples of transplant recipients, with urine samples providing higher sensitivity and blood samples providing higher specificity for probable BKAN. A positive relationship between BKV DNA levels in blood and impairment of renal allograft function has been shown, suggesting that viremic patients are at high risk for BKAN [27].
BKV viral load in the blood and urine has been demonstrated to be significantly higher in patients with biopsy-proven BKAN compared to those without BKAN [34]. In a study of 78 kidney transplant recipients, including ten patients who developed BKV viremia at a median of 23 weeks after transplantation, the mean BKV viral load in plasma was significantly higher in the five patients with BKAN than patients without histologic evidence of nephropathy (2 × 104 copies/ml vs 2 × 103 copies/ml, respectively; p < 0.001) [40]. Furthermore, the plasma BKV level increased over time to as high as 1 × 107 copies/ml in all patients who developed BKAN. BKV plasma viral load had 100 % sensitivity, 88 % specificity, 50 % positive predictive value, and 100 % negative predictive value for histologically proven BKAN [39, 40]. In another study, a BKV viremia threshold of >1.6 × 104 copies/ml had 100 % sensitivity, 96 % specificity, 50 % positive predictive value, and 100 % negative predictive value of diagnosing BKAN [39]. In this same study, a BKV viruria threshold of >2.5 × 107 copies/ml had 100 % sensitivity, 92 % specificity, 31 % positive predictive value, and 100 % negative predictive value for diagnosis of BKAN [39].
Molecular assays are the most common method for diagnosis of BKV infection in allogeneic HSCT recipients with hemorrhagic cystitis. BKV often is considered in the differential diagnosis of late onset hemorrhagic cystitis in HSCT recipients. The optimal test for detecting BKV in the urine is PCR for viral DNA. However, demonstrating BKV DNA by PCR alone has low specificity since even healthy individuals and HSCT recipients without hemorrhagic cystitis may shed and excrete BKV DNA in the urine. To improve the specificity, other features of reactivated BKV should be demonstrated including high peak BKV DNA (usually in the range higher than 109–1010 copies/ml or greater, a three log rise in BKV viral load from baseline that coincides with the onset of late onset hemorrhagic cystitis, or the presence of significant BKV viremia (usually higher than 104 copies/ml) [37]. If BKV DNA is not demonstrated by PCR in patients with hemorrhagic cystitis with decoy cells, other viral etiologies should be considered, such as adenovirus and CMV.
Molecular tests are the most common method for screening kidney transplant patients for BKV infection. The results of prospective studies indicate that BKV replication, as measured by BKV DNA qPCR tests of the urine, precedes BKV viremia by a median of 4 weeks [29, 30], and histologically proven BKAN by a median of 12 weeks [29]. Current guidelines recommend screening kidney transplant recipients for BKV infection by urine and/or plasma BKV qPCR at least once every 3 months during the first 2 years after transplantation [27, 41], and then annually until the fifth year after transplantation [27, 41]. In high-risk individuals, such as those who received intense immunosuppression for the treatment of acute rejection, other experts recommend monthly screening during the first 6 months after kidney transplantation [27, 41]. Using this strategy, at least 80–90 % of patients who develop BKAN can be identified before significant histopathology and functional renal impairment occurs [27, 41]. All patients with high-level urinary BKV viral load (>107 copies/mL) should have follow-up plasma BKV viral load testing [27, 41]. In patients with sustained plasma BKV viral load of >104 log 10 copies/ml for more than 3 weeks, a presumptive diagnosis of BKAN is made [27, 30]. The positive predictive value for BKAN in patients with high-level urinary BKV viral loads (>107 copies/ml or decoy cells) persisting for more than 2 months [30, 31, 35], or plasma BKV viral loads of >104 copies/ml is more than 50 % [27, 41]. Moreover, in patients with allograft dysfunction, the positive predictive value of plasma BKV viral load may exceed 90 % [42]. If definitive diagnosis of BKAN is sought in patients with suggestive urine and blood BKV viral loads, allograft biopsy and histopathology may be performed.
Molecular tests can be used to monitor response to treatment. BKV viral load testing is used for monitoring the response to antiviral therapy or reduction of immunosuppressive therapy in patients with BKV infection and disease [27, 41, 42]. An effective treatment response following reduction in immunosuppression, treatment with CDV or leflunomide, or allograft nephrectomy in kidney transplant recipients with BKAN is indicated by a decline in BKV viral load in the blood and urine, although the rate of decline over time is not uniform or defined [27, 41, 42]. A similar decline in BKV viral load should be seen following CDV or leflunomide therapy in HSCT recipients with late onset hemorrhagic cystitis [37].
The caveat in the clinical utility of molecular tests for the diagnosis and monitoring of BKV-associated disease is the lack of standardization (Table 47.2). The performance of these LDTs can vary widely due to differences in the specimen type used (for blood samples: whole blood or plasma; for urine samples: cell pellets, supernatants, or resuspended urine samples), the methodology, and the limits of detection, among others. Methodologies vary widely in terms of nucleic acid extraction, molecular amplification target gene (large T gene or VP-1 gene), quantitative calibrators, and detection reagents [43]. As a result, values obtained by different LDTs may not be comparable, which makes development of interpretive clinical guidelines difficult. The planned establishment of a WHO international quantitative standard for BKV should dramatically improve test standardization and may have a substantial impact on our ability to monitor and treat patients in a more uniform manner.
The Herpes Viruses
Cytomegalovirus
Description of Pathogen and Clinical Utility of Testing
Cytomegalovirus (CMV), a large, enveloped, double-stranded DNA virus belonging to the Betaherpesvirinae subfamily, is the most common herpes infection in SOT and HSCT recipients [44, 45]. A ubiquitous infection in humans, about 60–80 % of adults have serologic evidence of previous CMV infection [46–49]. Primary CMV infection may occur during infancy, preschool age, or sexually active years. Primary CMV infection in immunocompetent individuals often has a subclinical presentation, although it also may manifest with an infectious mononucleosis-like illness characterized by fever and lymphadenopathy. After the resolution of the primary infection, CMV becomes latent in various cells, with episodic subclinical reactivation throughout life that is controlled by a functioning immune system [46–49]. In transplant recipients, however, CMV reactivation and infection may result in a potentially severe clinical illness.
Transplant recipients may acquire CMV as a primary infection, reactivation, or reinfection (or superinfection) with a new strain [46–48]. Primary CMV infection occurs when a CMV-seronegative transplant candidate receives an allograft, hematopoietic stem cells, or blood products from a CMV-seropositive donor (CMV D+/R− transplant) [46–48]. Primary CMV infection is often characterized by severe illness due to the inability of immunosuppressed transplant patients to mount an effective immune response. Reactivation CMV infection occurs in a CMV-seropositive transplant recipient (CMV R+) when endogenous latent virus reactivates from latency. Reactivation of CMV often is associated with milder clinical illness among SOT recipients. However, reactivation of CMV may lead to severe and recurrent disease for allogeneic HSCT CMV R+ patients, especially if they receive hematopoietic stem cells from a CMV-seronegative donor (CMV D−). Reinfection or superinfection occurs when a CMV R+ patient receives an allograft from a CMV D+, and the infection is due to donor-transmitted virus. Genetic analysis demonstrates that the majority of CMV infection in CMV D+/R+ patients originates from donor-transmitted virus [50].
The clinical manifestations of CMV disease after transplantation can be classified either as CMV syndrome or tissue invasive disease. CMV syndrome, which occurs in approximately 70 % of CMV disease cases, is characterized by fever, arthralgias, and myelosuppression [47–49]. In 30 % of cases, CMV disease presents with end-organ involvement, such as interstitial pneumonia, hepatitis, esophagitis, gastritis, colitis, and rarely chorioretinitis [47–49]. In addition, CMV is associated with numerous indirect effects such as increased risk of allograft rejection, graft failure, and concomitant opportunistic infections due to bacteria, viruses, or fungi [47–49].
The incidence and severity of CMV disease in transplant recipients is influenced by the type of organ transplant, CMV status of the donor and recipient, and the overall level of immunosuppression [47–49]. The highest risk groups are lung and intestinal transplant recipients and allogeneic HSCT recipients, especially those who receive a haploidentical transplant and cells from unrelated donors. Individuals with no preexisting CMV-specific immunity, such as CMV D+/R− SOT patients, are at highest risk of CMV disease. CMV R+ patients who received allogeneic HSCT from a CMV D− are at high risk of CMV disease due to the lack of CMV-specific immunity in the transplanted hematopoietic stem cells. Symptomatic disease may occur in any transplant patient if there is severe suppression of the immune system, such as following treatment of rejection or GVHD.
Due to the negative impact of CMV disease on transplant outcome, prevention is a key component in the management of all SOT and HSCT recipients [45, 51]. The two approaches used to prevent CMV disease in transplant patients are antiviral prophylaxis and preemptive therapy. Antiviral prophylaxis is accomplished by the administration of antiviral drugs, most commonly oral valganciclovir, for a defined period of time to all patients at risk for CMV disease [45, 51]. The major disadvantage of this approach is delayed- onset CMV disease, which occurs in high risk CMV D+/R− SOT patients after they complete antiviral prophylaxis [52]. In preemptive therapy, transplant recipients are monitored using CMV PCR or antigenemia tests to detect early CMV replication; and antiviral treatment, usually valganciclovir or intravenous ganciclovir (GCV), is administered as soon as viremia or antigenemia is detected but prior to the onset of clinical symptoms [53]. The strategy of preemptive therapy is dependent on the availability of CMV NAT or antigenemia assays to guide treatment decisions (see discussion below) [2].
Available Assays and Interpretation of Results
Histopathology and Serology
Laboratory tests are essential to confirm the clinical suspicion of CMV disease in transplant patients. Histopathology with or without immunohistochemistry or in situ hybridization may be required to confirm tissue-invasive CMV disease. Because of the impaired and delayed ability of transplant patients to mount an effective immune response, CMV serologic testing to demonstrate IgM and IgG antibodies against CMV is rarely useful for the diagnosis of CMV disease after transplantation. Instead, serologic testing is used mainly prior to transplantation for CMV disease risk stratification. Depending on the donor and recipient CMV immune status, patients may be classified as high risk (e.g., CMV D+/R− SOT recipients) or low risk (CMV D−/R− patients) [45].
Viral Culture
Viral culture is a highly specific method that confirms the clinical suspicion of CMV disease. Viral culture can be done of blood and other body fluids such as respiratory secretions and cerebrospinal fluid (CSF). Viral culture of urine specimens is of low sensitivity and specificity for active CMV disease, since it often indicates urinary shedding, and therefore is not recommended for routine use in adult transplant recipients [2, 45, 51]. While viral culture of blood is highly specific and predictive of CMV disease and its severity, its major drawbacks are poor sensitivity and slow turnaround time [2]. The turnaround time for conventional culture results is between 2 and 3 weeks, thereby rarely affecting clinical care. The rapid centrifugation shell vial method has reduced the time for virus detection, with results obtained in 24–48 h, but this method still lacks sensitivity (compared to antigenemia or molecular techniques) [2]. Because of this, viral blood culture is no longer commonly used in clinical practice, although it remains helpful for end-organ viral detection in respiratory secretions and tissue samples.
CMV Antigenemia Tests
Antigenemia assays are based on detection of CMV proteins such as pp65 in peripheral leukocytes [2]. CMV antigenemia was used by many transplant centers for CMV surveillance and diagnosis because it is much more sensitive than viral culture [45]. Numerous studies have demonstrated the clinical utility of CMV antigenemia assays for rapid diagnosis of CMV disease, for surveillance of patients at high risk of infection (and to guide preemptive therapy), and for monitoring treatment response [54, 55]. However, the antigenemia assay is a labor-intensive test and lacks standardization. Since it relies on the presence of peripheral leukocytes, the assay must be performed within a relatively short period after sample collection and prior to leukocyte degradation in clinical samples [2]. The reliance on leukocytes may limit the applicability of the CMV antigenemia test in certain patient populations such as those with leukopenia, including HSCT recipients.
Molecular Tests for CMV Detection and Quantitation
Molecular testing methods have revolutionized the laboratory diagnosis of CMV disease in transplant recipients [2]. Laboratories have increasingly relied on NAT for CMV detection and quantitation, with the vast majority of CMV NAT being LDTs [56]. Commercial primers and probes are available as analyte specific reagents (ASRs). Most qualitative NATs that detect CMV DNA are difficult to interpret due to the potential detection of latent virus. Hence, the vast majority of CMV NATs rely on quantitative viral DNA detection [2, 56]. Assays that target RNA are more specific for active viral infection, although they suffer from modest sensitivity [57].
As with other quantitative assays described in this chapter, the major drawback to quantitative CMV NAT was the lack of standardization until recently [2, 3]. A wide range of factors may affect the performance characteristics of CMV NATs, including result accuracy and precision (Table 47.2). Difference in specimen type (i.e., whole blood, plasma, serum, leukocytes) is a common contributor to assay performance variability between laboratories. For example, NATs using whole blood are more sensitive than use of plasma samples, especially at lower viral load values, but less specific [58]. Nucleic acid extraction methods (e.g., manual vs automated, or liquid phase, magnetic bead or silica membrane/column), molecular amplification target gene (DNA polymerase gene, glycoprotein B gene, immediate early gene, major immediate early gene, or other CMV genes), and primer sequences and detection reagents (commercial vs noncommercial), quantitative calibrators (Roche, Acrometrix, Advanced Biotechnologies, and Qiagen), number of PCR cycles, and the range and limits of detection are some of the other factors that cause variability in the results of CMV NATs [43]. For example, the mean CMV viral load reported by laboratories that used Roche calibrators (4.32 log 10) was significantly lower than those that utilized Acrometrix (4.87 log 10, p = 0.0003), ABI (5.06 log 10, p < 0.0001), and Qiagen (5.03 log 10, p < 0.0001) calibrators [43]. Result variability using Roche calibrators were lower compared to Acrometrix and ABI calibrators [43]. Accordingly, the results of CMV NATs vary widely and are not directly comparable. The lack of standardization among the various CMV NATs was highlighted by a paper comparing the results of samples sent to 33 different laboratories across North America and Europe [3]. In this study, wide inter-assay variability in viral load was reported by the participating laboratories [3]. For example, one sample had a four-log difference in viral load reported from two laboratories [3].
In 2011, the World Health Organization developed an international quantitative CMV standard, with the goals of adoption by commercial manufacturers and individual laboratories for CMV NATs. The COBAS Ampliprep/COBAS Taqman CMV test (Roche) has been calibrated according to this international standard, and was approved by the US FDA for viral load monitoring of SOT patients with CMV disease [59]. This specific CMV test has a limit of detection of 137 IU/ml up to 9.1 × 106 IU/ml [56, 59]. Reporting of all quantitative CMV results in international units (IU) should allow portability of results (comparable results achieved irrespective of laboratory or method), assisting in care of individual patients and in the effort to develop uniform guidelines for treatment [56].
CMV NATs are the most commonly used method to establish the diagnosis of CMV disease in transplant recipients [45, 56]. Numerous studies established the clinical utility of LDTs and commercially available quantitative PCR assays for the diagnosis of CMV disease in transplant patients [45, 47, 48, 51, 60, 61]. Generally, transplant patients with CMV disease have higher viral load values than those with asymptomatic infection [53]. For example, a single-center study demonstrated that compared to those without detectable viremia, the risk of CMV disease is increased 50-fold in liver transplant recipients with viral loads greater than 2,860 copies/106 peripheral blood leukocytes [53]. However, the specific quantitative thresholds to signify clinically relevant disease from asymptomatic infection have been difficult to define, largely due to the lack of assay standardization noted above. Numerous authors have suggested that quantitative viral threshold values associated with active disease vary depending on the sample type (plasma vs whole blood vs leukocytes), type of transplant (lung vs kidney, and SOT vs HSCT), the overall net state of immunosuppression (T-cell depleted vs non T-cell depleted), whether CMV infection is primary vs reactivation, and the assay used for detection, among other factors. Moreover, there may be cases of compartmentalized (or localized) tissue-invasive CMV disease that have no detectable level of viremia. In these cases, demonstration of CMV in tissue by histopathology may be required [47, 48, 53].
CMV NATs are useful in predicting the risk of CMV disease in transplant recipients [45, 56]. Using CMV PCR assays, transplant patients are monitored for evidence of CMV replication so that antiviral therapy can be administered prior to the development of clinical symptoms [53, 62]. This is the principle behind the “preemptive therapy” approach to CMV disease prevention, which is the most common strategy for the prevention of CMV disease in allogeneic HSCT recipients. Preemptive therapy also can be used in SOT recipients at moderate risk of CMV disease, such as CMV R+ kidney and liver transplant recipients. Using this approach, blood samples for CMV detection are collected at least once weekly during the first 12 weeks (or 100 days) after transplantation; some centers may monitor highest risk allogeneic HSCT recipients more frequently (e.g., twice weekly). Early studies evaluating the clinical utility of qualitative CMV PCR using cell-based samples demonstrated high sensitivity, poor specificity, and poor positive predictive value [2].
Several more contemporary approaches have been developed to improve the clinical specificity of CMV NAT, including the use of quantitative PCR assays, detection of CMV DNA in plasma (instead of leukocytes), and detection of CMV messenger RNA (mRNA) rather than DNA. Detection of CMV DNA in plasma rather than in leukocytes provides better correlation with clinical disease [2]. Quantitative CMV NAT methods have the potential to define a viral threshold that distinguishes asymptomatic infection from active CMV disease. For example, in a single center study that evaluated 97 liver transplant recipients by quantitative CMV PCR testing, a viral load threshold between 2,000 and 5,000 copies/ml of plasma was defined as the optimal cut off for predicting CMV disease with a sensitivity of 86 %, specificity of 87 %, positive predictive value of 64 % and a negative predictive value of 96 % [62]. However, for reasons discussed above, the lack of assay standardization has limited the generation of a viral load threshold that is widely applicable across populations and laboratories.
CMV viral load testing is useful in assessing therapeutic response in patients with CMV disease [45, 56]. Three antiviral drugs are US FDA-approved for the treatment of CMV disease: GCV, CDV, and foscarnet (phosphonoformic acid [FOS]); of these three, GCV is the most widely used in clinical practice. Current guidelines recommend that the duration of treatment of CMV disease should be guided by the clinical resolution of symptoms and clearance of the virus from the blood [44, 45]. Accordingly, CMV viral load testing is performed weekly to document virologic response and clearance during antiviral treatment. Several studies have reported > 90 % or about one log reduction in viral load during the first 1–2 weeks after initiation of antiviral therapy in the vast majority of patients [63, 64]. In a minority of patients, the viral load decline may be delayed for up to 2 weeks. Moreover, the rate of viral load decline varies by individual patient, as influenced by the underlying immunosuppression, preexisting virus-specific immunity, and potentially viral strain variation [65, 66]. Patients with low pretreatment viral loads will generally achieve an undetectable viral load sooner than those with high pretreatment viral loads [59, 64]; hence, more prolonged antiviral therapy generally is given for patients with higher initial viral loads. Thus, molecular tests allow an individualized approach to management. In general, antiviral therapy is continued until the CMV viral load is below the limit of detection [51, 59]. Discontinuation of antiviral treatment in patients with detectable viremia is a risk factor for CMV disease relapse [67]. However, an overly sensitive CMV viral load test may lead to over-treatment if the assay can detect “non-clinically relevant” virus [61].
CMV viral load testing is a useful marker of CMV disease relapse. The optimal duration of antiviral treatment for CMV infection and disease, as discussed above, should be individualized based on clinical and virologic resolution of infection. Hence, sequential monitoring of CMV viral load after initiation of therapy allows the identification of patients at risk of recurrent infection. Up to 30 % of transplant patients treated for CMV infection and disease will develop relapse of viremia or clinical symptoms after discontinuation of antiviral therapy. In clinical practice, CMV relapse is heralded by the reappearance of CMV DNA in the blood following initial successful viral load suppression. Patients generally are monitored by CMV viral load testing weekly for up to 4 weeks following an antiviral treatment course to monitor for relapse. Retreatment with intravenous GCV or oral valganciclovir is generally effective for treatment of relapse.
CMV relapse is most common in patients with severe immunosuppression and without CMV-specific immunity. Early laboratory predictors of CMV relapse are a high peak CMV viral load and a slow rate of decline in CMV viral load (also known as viral decay) [62, 68, 69]. In a study of 52 SOT recipients with CMV disease who were monitored with the Amplicor CMV Monitor test (Roche) [68, 69], the time to clearance of CMV DNA from plasma was 33.8 days in the 12 patients with relapsing CMV disease compared to 17.2 days in the group without recurrent disease (p = 0.002). The viral load half-life with treatment was 8.8 days compared to 3.2 days (p = 0.001) in the group with and without recurrent disease, respectively [62]. Moreover, those with very high pretreatment viral load values had higher risk of CMV relapse [67]. In a prospective study of 24 SOT patients with CMV infection or disease who received a 14-day course of IV GCV, the CMV viral load prior to the initiation of antiviral therapy was statistically higher in eight patients who had CMV relapse compared to the 14 who did not relapse (80,150 copies/106 leukocytes vs 5,500 copies/106 leukocytes, respectively, p = 0.007) [67]. As discussed above, transplant patients who discontinue antiviral therapy with ongoing viremia have a higher risk of clinical relapse, compared to those who have attained viral load suppression, even if they have resolution of clinical symptoms [67]. In this study, those who had CMV infection relapse had a detectable viral load at the end of treatment (mean 18,800 copies/106 leukocytes), while viral load was undetectable in the non-relapsing group [67].
CMV Drug Resistance Testing
Failure of viral load to decline to an undetectable level, or a rise in viral load after an initial decline, following initiation of antiviral therapy suggests potential drug resistance [45, 51, 70]. Risk factors for CMV drug resistance are (1) prolonged exposure to antiviral drugs, (2) severe immunosuppression, (3) lack of CMV-specific immunity (i.e., CMV D+/R−), and (4) suboptimal antiviral drug concentrations [45, 51, 70]. Fortunately, resistance to GCV and other drugs is uncommon, with rates estimated at less than 5 % of all CMV disease cases [45, 51, 70].
CMV drug resistance can be confirmed by either phenotypic or molecular (genotypic) testing [71]. Phenotypic assays rely on analysis of growth characteristics of viral isolates in the presence of different concentrations of antiviral drugs and therefore require viable virus. Phenotypic assays are biologically more relevant for detection of drug resistance. However, phenotypic assays are labor intensive with subjective test result interpretation, and require at least 1 month to obtain results, which is generally not timely for clinical care.
Molecular assays can detect the presence of nucleotide sequence changes associated with drug resistance [71]. Genotypic results generally correlate with clinical drug resistance and provide rapid results that can guide clinical therapeutic selection. Drug resistance is indicated by mutations in the UL97 gene (which encodes viral kinase) and/or the UL54 gene (which encodes the CMV DNA polymerase) [71]. UL97 mutations are more common and confer resistance to GCV, while UL54 mutations are less common but confer resistance to any or all of the three anti-CMV drugs (GCV, FOS, and CDV). GCV resistance mutations are most frequently mapped to the UL97 gene within codons 460 and 520 and either point mutations or deletions within the codon range 590–607 [71]. One caveat for genotypic assays is the presence of some normal baseline sequence variability in the UL97 and UL54 genes in drug-sensitive CMV strains [71].
Epstein Barr Virus
Description of Pathogen and Clinical Utility of Testing
EBV is an oncogenic gamma-herpesvirus that causes B-cell proliferation in humans [72]. A common infection in humans, EBV frequently is acquired by exposure to infected body fluids, such as saliva. In healthy individuals, primary EBV infection can be asymptomatic or can cause infectious mononucleosis, which is characterized by fever, sore throat, and lymphadenopathy. Following primary infection, EBV establishes lifelong latency in various organs which serve as reservoirs and vehicles for transmission to susceptible EBV-seronegative transplant recipients.
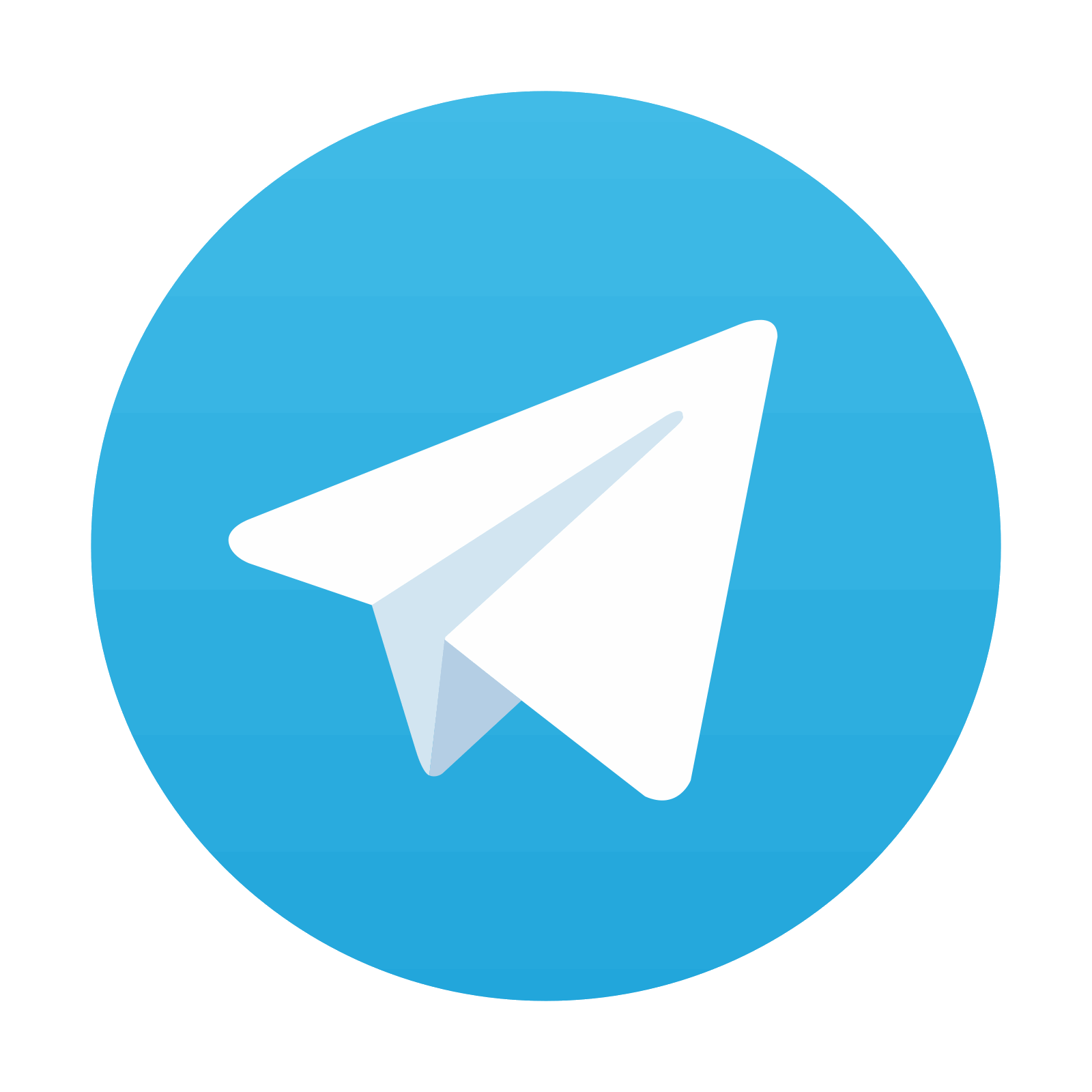
Stay updated, free articles. Join our Telegram channel
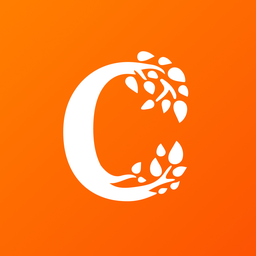
Full access? Get Clinical Tree
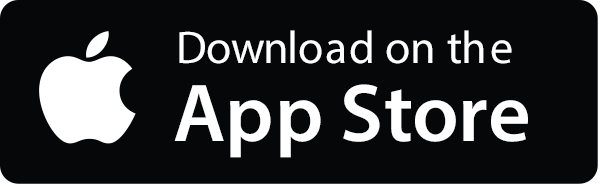
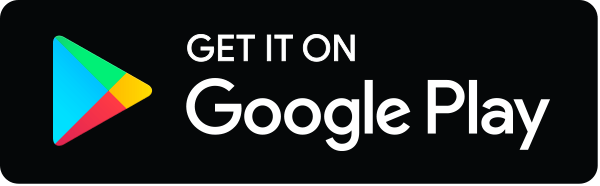