Figure 14.1 Nanosystems that exist in nature (not to scale). Left to right: an insulin hexamer (3.5 × 5 nm), a low-density lipoprotein (LDL) particle (28 nm) and a parvovirus (20–25 nm). LDL with its apolipoprotein ligand is a natural carrier of cholesterol to rapidly dividing cells; viruses are efficient transfection agents.
Although the descriptor nanotechnology came to the fore in the late 1980s it has a somewhat longer pedigree in pharmacy, since, in fact, Peter Speiser and his colleagues1,2 at ETH in Zurich prepared and investigated ‘nanoparts’ and ‘nanocapsules’ a decade earlier.3 Surfactant micelles (see Chapter 5) have diameters of some 1–3 nm and have been studied for more than 70 years as solubilisers of poorly water-soluble drugs. Microemulsions (see section 6.4.5), a name coined in 1959,4 are more correctly named nanoemulsions and are used in pharmacy, as in the ciclosporin concentrate Neoral, which on dilution forms droplets in the size range 10–100 nm (see section 6.4.6).
Typical nanosized pharmaceutical carrier systems therefore include polymeric or lipidic nanoparticles, nanoemulsions (microemulsions), dendrimers, fullerenes and carbon nanotubes as well as hybrid systems and the smallest liposomes. Some poorly soluble drugs can be prepared as nanosuspensions. Some systems are represented in Fig. 14.2.
Figure 14.2 Typical nanocarriers and imaging systems in the size range 1–100 nm (not drawn to scale), which will be increasingly used in pharmacy as carriers of drugs and diagnostic agents: shown here in comparison with molecules, viruses, bacteria, cancer cells and a full stop in printing.
Reproduced with permission from McNeil SE. Nanotechnology for the biologist. J Leukoc Biol 2005;78:585–593.
Clearly a main focus in pharmaceutical nanotechnology is how to characterise nanosystems. This includes investigation of drug loading capacity, drug release rate and the chemical and physical stability of both drug and carrier (Fig. 14.3). Particle size is of course a key property, and surface charge and character (whether the surface is hydrophilic or hydrophobic) are other defining properties. Shape, flexibility or elasticity of particles can also be factors of importance. Biological evaluation of nanosystems involves knowledge of the absorption, distribution and excretion of both the drug and the carrier along with its load of encapsulated or attached drug. The mode and speed of the biodegradation of the nanoparticle are also required information.
Figure 14.3 Pharmaceutical nanotechnology’s main areas of focus, namely, applications, manipulation and processing, physicochemical characterisation and biological evaluation. An understanding of the relevance of the physicochemical properties of these systems and biological barriers and environments they encounter is essential. Absorption, distribution, metabolism and toxicology refer to both the drug once released from the delivery system and the delivery system itself, the two being intertwined.
14.2 Rationale for nanoparticles for drug delivery and targeting
The rationale for: (a) the encapsulation of drugs in nanoparticles or (b) in some cases the adsorption of active agents on to the surface of nanoparticles is so that:
1. the active molecules are protected from the external environment until such time as they are released. Once released, any drug will behave as free drug molecules, although being transported by the nanocarriers, the drug may have been deposited in the liver and spleen by the carrier
2. it is the nature, destination and fate of the nanoparticles, rather than the characteristics of the drug, that determine at least the initial fate of the drug because the body ‘sees’ the carrier rather than the drug
3. the pharmacokinetics of the active is modified by its slow release from the carrier and the carrier’s trajectory, which may lead to reduction in toxicity.
It is important to distinguish between the pharmacokinetics of drug delivered in the carrier and the kinetics of the particles themselves, a subject that is termed particokinetics.5 Size is particularly important in determining the ability of nanoparticles to access sites that are out of bounds to larger particles. There is, however, a continuum of diameters and one of the great challenges in drug delivery and targeting is the production of nanosystems with narrow size distributions. Nature achieves this, as we have seen in the case of low-density lipoproteins, which have a mean diameter of 26.7 nm and a size range of 25.8–28.2 nm in young healthy females and 25.5–28.1 nm in males.6 Small size at the limit where the drug molecules approach the size of the carrier (as with dendrimers in the 2–10 nm range) means a carrier/drug ratio of 1. Some nanoparticles, such as drug nanocrystals, on the other hand, consist virtually only of drug, as discussed in section 14.6.
One pharmaceutical problem is to design and formulate systems that are stable in vitro but can also survive their dilution in blood, and the mixing and shearing forces in flow. There are many changes in the particle environment as it passages through tissues, one of which is a change in surface characteristics brought about by opsonin adsorption, a precursor of uptake by the reticuloendothelial system and hence loss from the circulation.
14.3 Particle structures
To utilise fully the potential of nanotechnology a goal of selective delivery of drugs to cells, organs and tissues has been pursued. The general features of targeting systems are drawn in Fig. 14.4, which summarises the complex structural nature of such a nanoparticulate system comprising eight aspects of design:
1.nature of the interior space
2.nature of the shell
3.nature of the surface
4.interior payload levels
5.shell payload level
6.surface payload
7.surface coating
8.targeting ligands.
It may be that the particle is solid, formed from a single polymer without a shell. What is then important is the distribution of the drug throughout the system.
Figure 14.4 General elements of nanoparticles designed for targeting, using targeting ligands to interact with appropriate receptors on target cells or tissues. Surface coatings are used to modify nanoparticle behaviour in vivo. Interior, surface or shell-based payloads with the characteristics of the nanoparticle surface and the nature of the particle interior and shell, if present, are other factors in design and characterisation.
Reproduced with permission from Mieszawska AJ et al. Multifunctional gold nanoparticles for diagnosis and therapy of disease. Mol Pharm 2013;10:831–847.
14.4 The primacy of particle size
Nanotechnology is defined to a large extent by the size (or more usually, the size range) of the systems used. Only some systems, such as dendrimers, because they are synthesised as discrete chemical entities, have for any particular type a single size, i.e. they are monodisperse. Many other nanoparticle preparation techniques result in a particle size distribution. It is, however, possible to prepare monodisperse systems as with polystyrene and other commercially available systems which are often used as controls or models in experiments.
Small size brings with it both advantages and disadvantages. Rates of release may be rapid, because of the large surface area per unit particle weight and because diffusional path lengths within the particles are small. Particle size influences many of the properties and behaviour of nanoparticles, as indicated in Fig. 14.5.
Figure 14.5 Particle size and its bearing either directly or indirectly on the behaviour and fate of nanoparticles. For examples see text.
|
How can the nanocarriers be designed to release drug only when at a target site? The system could be activated once it had tracked to its destination, for example by elevation of local temperature, making use of enzymatic reactions, or changes in local pH, or by an external stimulus. It should be noted that the circulation times in the body of nanoparticles from a single administration vary depending on the frequency of individual particle uptake into the target. See section on stochatic processes (14.7.4) which discusses the importance of the stochastic nature of extravasation and the consequences for circulation times. |
Key parameters
- Drug release: the larger surface area per unit weight of nanoparticles leads to potentially high release rates compared to the same weight of larger particles, as solvent penetration will be a function of nπr2, where n = number of nanoparticles per unit weight and r is the particle radius.
- Oral uptake: although we state in Chapter 8 that drugs must be in their molecular form to be taken up by epithelial cells, there is a mechanism for oral particle uptake. The main site involves the membranous epithelial cells (M cells) of the gut-associated lymphoid tissue. Smaller particles (i.e. those 5–150 nm) with the appropriate surface properties are taken up more readily than the equivalent larger particles (>300 nm), and above a certain size (around 500 nm) particles will not be absorbed, at least not intact. The oral delivery of large labile molecules such as insulin is being pursued in spite of 90 or more years of failed experiments, but low uptake of carriers and the sometimes the very low capacity of the carriers for such molecules make this a daunting task if the nanoparticle approach is used for insulin and similar molecules.
- Flow in capillaries: once injected, nanoparticles flow in the blood and particle diameter is clearly important. Flow is a complex phenomenon, as discussed in Chapter 6; it depends on the ratio of particle diameter to the diameter of the capillaries, on bifurcations of the capillary network, particle flexibility and particle jamming (see below and Fig. 14.6).
- Embolisation: nanoparticles are too small to block blood vessels and capillaries, but if they aggregate then such blockage (embolisation) can occur. The process of particle ‘jamming’ can also occur (Fig. 14.6), which hinders free particle movements such as their escape from the circulation and passage though the extracellular matrix or porous media.
Figure 14.6 A simple illustration of particle jamming at the juncture of two vessels during particle flow. The phenomenon depends on the nature of the particles, their diameter in relation to the diameter of the capillaries and their branches, and the surface properties and flexibility or elasticity under pressure.
- Surface erosion is involved in many biodegradation and drug release processes, and will be a function of surface area of the nanoparticles and of course dependent on the nature of the polymer, lipid or other material used to fabricate the system.
- Adsorption of ligands: adsorption of proteins, whether derived from the blood or deliberately attached (e.g. polyethylene glycols) to alter the surface properties of nanoparticles. The number of ligands per particle will depend on the available surface area, and the area/molecule of the adsorbing molecules, the charge and hydrophobicity of the surface.
- Endocytosis (uptake of particles by cells) is size-dependent.
- Extravasation (escape of particles from the circulation into target cells or tissues) occurs more readily with small particles. Figure 14.7 shows a representation of the escape of nanoparticles from the circulation in the region of tumours where the vasculature is more porous than normal. The process of the potential route of particle tumour uptake by this mechanism has been termed the enhanced permeation and retention effect, but its relevance to many systems has been questioned. Further proof of the range of applicability is required.
Figure 14.7 Diagrammatic representation of the process of extravasation of nanoparticles in the vicinity of tumours; in normal tissue particle escape is limited.
- Physical (colloid) stability is dependent on radius, the nature of the material, its charge and the presence of any adsorbed molecules whose molecular weight and configuration on the surface are crucial (as discussed in Chapter 6).
|
To what extent can the original properties of a particle designed with surface groups and ligands be retained in vivo? |
In Fig. 14.8 the dynamic processes that occur at the surface of intravenously administered nanoparticles are illustrated.
Figure 14.8 Illustration of the dynamics of protein adsorption on to the surface of nanoparticles in the blood. Much depends on the original nature of the particle surface, but a primary corona of biomolecules (light blue) first adsorbs, followed by biomolecules (dark blue and pale blue) with a greater affinity and so on. The surface thus evolves with time.
Reproduced from Monopoli MP et al. Nature Nanotech 2012;7:79–786.
- Diffusion is vitally important in the translocation of nanoparticles and their transport through mucus and tissues, as well as in tumours and other targets. In a simple fluid medium of viscosity
the influence of particle radius, r, on diffusion coefficient, D, can be recognised from equation (14.1).
where k is the Boltzmann constant and T is temperature.
Movement in complex media, e.g. cells and gels, will be represented by more complex equations, as given in section 14.7.
As shown in Fig. 14.9:
- The compatibility of drug and carrier system is vital to the even distribution of drug throughout the nanoparticle and to avoid premature release that occurs when the drug migrates to the particle surface.
- The loading of the drug into the particles depends on the nature of the drug and the nature of the carrier material.
- The intravenous route results in mixing of the nanoformulation with blood, perhaps particle adsorption on to erythrocytes, opsonisation (which is the adsorption of the proteins or opsonins on to the surface of the nanoparticles which then cause the particles to be recognised as foreign and taken up by the liver and spleen), partial escape from the circulation by extravasation and interaction with the receptor before a cascade of events such as internalisation and diffusion with tissues.
Figure 14.9 A schematic of issues in the formulation and use of nanoparticles as targeted medicines.
Nanoparticles can be administered by a variety of routes, including the ocular, oral, intravenous, intraperitoneal, intraluminal, subcutaneous, intramuscular, nasal, pulmonary and intratumoral routes. Sometimes nanoparticle-based delivery depends not on any single property. With ocular delivery to the cul-de-sac of the eye, nanoparticles can prolong the action of a drug both by slow-release characteristics and by slowing the loss via the punctae when compared with a solution. The adhesive qualities of some nanosystems can also enhance site-specific activity.7 Bioadhesive nanoparticles have been advocated to assist adhesion of the carriers to the mucosa and thus increase the probability of arrest, as well as to provide time for the transfer of drug from the carriers or the particles themselves into the gut wall. The importance of the lymphoid tissue in the gut, the gut-associated lymphoid tissue, is crucial to the understanding of some of these possibilities. The M cells and lymphoid tissues of other anatomical regions can be important in particle delivery; for example, the nasal lymphoid tissue, the bronchial tissue and other sites (omentum) provide the possibility of access of particles in small quantities, which may be sufficient for immunisation with vaccines.
14.5 Physicochemical characterisation of nanosystems
The key physicochemical properties of nanoparticles are listed in Table 14.1 under two headings: those of importance in relation to use, and those important in relation to their preparation and properties.
Table 14.1 Key physical and chemical properties of nanoparticles as drug carriers
In relation to use |
Surface area |
Shape |
Drug release rate |
Biodegradation processes, including rate of degradation |
Diffusion (e.g. in tissues and mucus) |
Aggregation and flocculation (in vitro and in vivo) |
Adsorption (e.g. to biological and polymer membranes) |
Deposition (e.g. on surfaces) |
Rheology (e.g. in blood) |
Elasticity (to aid progress in capillary networks) |
In relation to preparation and properties |
Drug–polymer interactions and compatibility (e.g. important in loading drug into systems) |
Polymer–polymer interactions (e.g. when two polymers are used to fabricate particles) |
Hydrophobicity and hydrophilicity of surfaces |
Surface charge |
Surface adsorption of macromolecules |
To be successful therapeutically, systems must have optimal size and surface characteristics, high loading capacity and loading efficiency, a high degree of chemical and physical stability, as well as appropriate release characteristics at the target site and be biodegradable and biocompatible.
Not all drug (active)–polymer (carrier) combinations can be readily formulated, sometimes because of the low affinity of the drug for the carrier or indeed incompatibility between drug and carrier. For example, protein drugs do not always mix with polymers without phase separation. (Chapter 7 has a discussion of some of these issues.) Formulations in which drug and carrier material phase-separate (that is, do not produce an isotropic mixture) or whose production process leads to a movement of drug towards the surface of the particles usually demonstrate burst (initial fast) release effects. Size polydispersity can also lead to ambiguous results, as it complicates the achievement of targeting because particles of different sizes have different fates.
14.5.1 Hard and soft systems
While size defines all nanosystems, they can differ in their material properties, their chemistry and their physics. It is useful, for reasons that are apparent in relation to movement of nanoparticles in vivo, to divide nanosystems into two types, namely ‘hard’ and ‘soft’.
- Soft systems include nanoemulsions and polymeric micelles.
- Hard systems, which are neither flexible nor elastic, include polymeric nanoparticles, nanosuspensions or nanocrystals, dendrimers and to an extent carbon nanotubes.
Hard systems may block spaces and fenestrae that have the same dimensions as the particles, whereas soft systems can by definition deform and reform (erythrocytes and many liposomes fall into this category). They are thus better able to navigate constricted capillary beds and tissue extracellular spaces.
|
|
14.6 Preparing nanoparticles
There are a large number of methods to prepare nanoparticulate systems, as can be seen from Table 14.2. These depend to a large extent on the material that will form the basis of the carrier, which can include polymers, proteins, metals (gold) or ceramics.
Table 14.2 Methods of manufacturing polymeric or protein nanoparticlesa
Method | Relevant physical events |
Solvent displacement | Mixing of solvents to cause the material to come out of solution |
Salting out | Reducing solvency: as above, but using another component to salt out the material |
Emulsion diffusion | Diffusion of solvent from emulsion droplets, leaving behind the nanoparticle material |
Emulsion-solvent evaporation | Evaporation of solvents: Material in the droplets is revealed by removing solvent |
Supercritical fluid technology (SCT) | Solvent phase behavior: utilising SCT to produce nanoparticles |
Complexation, coacervation | Macromolecular interactions to create a complex mixture of e.g. polymers in the micro or nano size range |
Reverse micellar methods | Solubilisation of monomers in inverse micelles and polymerising the monomers by a variety of techniques |
In situ polymerisation | Growth of polymers in suspension |
Synthesis (e.g. dendrimers) | Covalent growth from a core molecule, by layer addition of linking groups |
aSome of the above methods are outlined in the text. A fuller account can be found in Florence AT. Pharmaceutical aspects of nanotechnology. In: Florence AT, Siepmann J (eds) Modern Pharmaceutics, 5th edn, vol. 2, chapter 12. New York: Informa Healthcare; 2009.
14.6.1 Solvent displacement method
The method of solvent displacement (Fig. 14.10) is perhaps the simplest means of preparing polymeric nanoparticles. The polymer is dissolved along with the drug to be encapsulated in commonly used water-miscible solvents – ethanol, acetone or methanol. The polymer solution is then diluted in the presence of stabilisers such as poly(vinyl alcohol) or polysorbate 80, and forms stabilised nanoparticles with narrow size distributions.
Figure 14.10 A summary of the solvent displacement method. The poly(vinyl alcohol) (PVA) or polysorbate 80 stabilises the particles as they precipitate following the dilution of the non-aqueous solvents. The rapid diffusion of solvent from the polymer phase allows rapid formation and hence a small size distribution.
14.6.2 Salting-out method
A variant on the above technique includes a polymer plus a drug dissolved in a water-miscible organic solvent which is added to a stirred aqueous gel containing a salting-out agent and a stabiliser. The polymer–drug combination is salted out as nanoparticles.
14.6.3 Emulsion-diffusion method
A different approach is illustrated in Fig. 14.11. A partly water-miscible solvent in which the drug and polymer are dissolved forms an emulsion on dilution in an aqueous phase containing a stabiliser. Because of the partial miscibility of the first solvent, it diffuses from the dispersed drops into the bulk to provide polymer nanoparticles containing drug.
Figure 14.11 Schematic of the emulsion technique wherein the drug and polymer are dissolved in a partly water-miscible solvent. This is then added to an aqueous phase in the presence of surfactant or other stabiliser. Emulsion particles are formed. The solvent, being partly miscible in the water phase, diffuses from the emulsion droplets to form nanoparticles.
14.6.4 Emulsion-solvent evaporation method
Figure 14.12 illustrates a different approach with the emulsion-solvent evaporation technique. It involves the dissolution of the drug–polymer mix in an organic solvent that is immiscible with water, so that on addition to an aqueous phase an emulsion is formed in the presence of emulsifiers. On heating, the internal organic phase can be evaporated in a controlled fashion leaving the polymer–drug nanoparticles, whose size is dependent on the nature of the emulsion.
Figure 14.12 A process similar to that in Fig. 14.11. The polymer/drug organic solution is emulsified in water as an oil-in-water system, or the polymer and drug are dissolved in an aqueous phase, which when added to an organic phase forms a water-in-oil emulsion. The internal phase in both cases is evaporated, leaving the polymer–drug particles.
In a variant of this process the drug and the polymer (or protein such as albumin) are dissolved in water, and then added to an organic phase. A water-in-oil emulsion is formed initially, from which the water is removed by evaporation. The oily phase is later ‘washed’ off.
14.6.5 Using supercritical fluid technology
A supercritical fluid is a substance at a temperature and pressure above its thermodynamic critical point (Tc) (Fig. 14.13a). In this state it can diffuse through solids like a gas, and dissolve materials like a liquid. Carbon dioxide and water are the most commonly used supercritical fluids. Supercritical fluid technologies deserve a special mention as they are less commonly applied at a laboratory scale in the preparation of nanoparticles. A drug and polymer are dissolved in an organic solvent or carbon dioxide. Under certain conditions of pressure and temperature (Fig. 14.13(a)) the liquid phase is transformed into the supercritical state, which for CO2 occurs at pressures >74 bar (atmospheric pressure = 1.013 bar (101.3 kPa)) and temperatures >31°C (Tc). The rapid expansion of this supercritical solution on exposure to atmospheric pressure causes the formation of microspheres or nanospheres.
Figure 14.13 (a) The pressure–temperature phase diagram of CO2 showing the Tc and Pc at the supercritical region. (b) Schematic of the process of producing nanoparticles using supercritical fluid technology. (See, for example, Byrappa K et al. Nanoparticle synthesis using supercritical fluid technology – towards biomedical applications. Adv Drug Deliv Rev 2008;60:299–327.)
14.6.6 Coacervation and complexation techniques
A coacervate is a gel-like liquid state of matter that can be treated to produce solid microparticles or nanoparticles. There are several possibilities: one is complex coacervation, in which positively charged macromolecules are mixed with negatively charged macromolecules. Under certain conditions of temperature and relative concentration and ionic strength, there will be a phase separation whereby a coacervate forms (Fig. 14.14). In simple coacervation with single macromolecules of either charge, solvent change and salting out create the conditions for the formation of coacervate particles.
Figure 14.14 A phase diagram of PEGylated chitosan as a function of tripolyphosphate (TPP) concentration showing the region where ‘nanogel’ coacervate nanoparticles form.
Reproduced with permission from Wu Y. Chitosan nanoparticles as a novel delivery system for ammonium glycyrrhizinate. Int J Pharm 2005;295:235–245. Copyright Elsevier 2005.
14.6.7 In situ polymerisation
In this mode of synthesis of polymeric nanoparticles (e.g. of polybutylcycanoacrylates), monomeric materials (e.g. butylcyanoacrylate) are dispersed in a suitable solvent and emulsified. An initiator is generally added to the system and the monomers condense to form a polymeric matrix. In the case of the alkyl cyanoacrylates, no initiator is required as the aqueous medium itself acts as the initiator of polymerisation.
14.6.8 Synthetic and semisynthetic processes: dendrimers and carbon nanotubes
Dendrimers are synthesised ‘generation’ by ‘generation’ from a multivalent core molecule, as demonstrated in the model in Fig. 14.15. Carbon nanotubes are obtained from soot and processed to form single-walled nanotubes or multiwalled nanotubes, shown in Fig. 14.16.
Figure 14.15 Scheme of the stepwise synthesis of dendrimers to form spherical or quasi-spherical nanocarriers. Top left: second-generation polyamidoamine (PAMAM) dendrimers; bottom right: the molecular weights and number of end-groups on second- to sixth- generation PAMAM dendrimers. Chapter 7 has more details.
Model, Deutsches Museum, Munich, 2009.
Figure 14.16 Representation of a single-walled carbon nanotube (CNT). In the photomicrograph on the left the native state of carbon nanotubes is seen, and on the right, double-walled nanotubes at the same magnification. The surface of the CNTs can be altered through adsorption of desired molecules or by covalent attachment of molecules for the purpose of stabilisation (for example, to prevent aggregation) or by addition of drug molecules or targeting moieties.
Reproduced with permission from Mérard-Moyen C et al. Functionalized carbon nanotubes for probing and modulating molecular functions. Chem Biol 2010;17:107–115. Copyright Elsevier 2010.
14.6.9 Polymeric fibres and tubes
The production by electrospinning of polymeric tubes and fibres with diameters in the nanometre size range was discussed in Chapter 7. These are being studied for a variety of delivery challenges.
14.6.10 Drug nanocrystals
Nanocrystals are formed by a variety of techniques, including high-pressure homogenisation, sonochemical processes (e.g. for azithromycin8), continuous precipitation techniques, by using microemulsions as templates (as emulsions can be used to prepare microparticles) or milling in appropriate media. The purpose is to enhance the surface area of poorly soluble drugs with the prospect of enhanced solubility and absorption. Figure 14.17 shows a scanning electron microscopy photograph of paclitaxel nanocrystals, one of many drugs that have been formulated as nanosuspensions.
Figure 14.17 Scanning electron microscopy photograph of paclitaxel nanocrystals.
Reproduced from Hollis CP et al. www.cntc.uky.edu.
Nanocrystals of poorly water-soluble drugs are of increasing importance in pharmacy as their greatly reduced particle size increases the surface area per unit weight and hence increases the rate of dissolution of the drug, all things being equal. The Noyes–Whitney equation (see Chapter 1) relates the change of weight, dw, with time, dt:
where A is the surface area of the material, D is the diffusion coefficient, δ is the diffusion layer thickness and cs is the saturation solubility, c being the concentration of agent in the solution at any given time t. There may be a degree of supersaturation, but the equilibrium solubility is unaffected by particle size until very small particle sizes are reached, as shown in Fig. 14.18. The critical value depends, as can be seen, on the surface energy of the material in question. The combination of increased intrinsic solubility and increased surface area has the potential to change solution, and hence absorption properties dramatically. If the absorption of the drug is dissolution rate-limited this will assist in the extent of absorption; enhanced solubility has the potential to increase concentration gradients across the biological membrane.
Figure 14.18 The solubility enhancement S/S0 as a function of particle diameter for three hypothetical compounds with surface energies of 50, 75 and 100 mN m−1. It can be seen that where S0 is the solubility of a large crystal, and S is the solubility of the crystal of a specific size, the solubility enhancement (S/S0) does not increase significantly until particle diameters reach below around 75 nm.
Reproduced from Kipp JE. The role of solid nanoparticle technology in the parenteral delivery of poorly water-soluble drugs.Int J Pharm 2004;284:109–122. Copyright Elsevier 2004.
Figure 14.19 demonstrates the effect on the plasma level versus time plots of danazol of a nanosized suspension over a conventional suspension. It can be seen that nanocrystal formation leads to results equivalent in this case to the effect of solubilisation in a cyclodextrin.
Figure 14.19 Danazol is a drug with a low solubility of circa 10 μg mL−1. Here plasma drug levels are plotted as a function of time after administration of three forms of the drug, including a nanoparticulate dispersion and a hydroxypropyl-β-cyclodextrin complex, using a suspension with a mean particle size of 10 μm as a comparator.
Reproduced from Liversidge GG and Cundy KC. Particle size reduction for improvement of oral bioavailability of hydrophobic drugs: I. Absolute oral bioavailability of nanocrystalline danazol in beagle dogs. Int J Pharm 1995; 125: 91–97.
If nanocrystals are used as suspensions clearly as they are colloidal they must be stabilised, for example by surface adsorption of non-ionic surfactants. The ratio of any stabiliser to the drug content must be carefully titrated, as in any stability study. However, nanocrystals can be used not only as suspensions but also as freeze-dried powders for reconstitution; they can be incorporated into liposomes, used as aerosols, incorporated in gels or in microspheres, adsorbed on to microparticles, dispersed in soft gelatin capsules or used as mini-depot tablets.
|
solvent displacement salting out emulsion-diffusion emulsion-solvent evaporation use of supercritical fluid technologies coacervation and complexation techniques in situ polymerisation nanocrystal formation by a variety of techniques of size reduction. |
14.7 Challenges in targeting
There are many potential advantages and exciting opportunities in deploying nanocarriers to achieve end-points that the drugs themselves cannot achieve unaided. This would include enhanced accumulation in particular pathological sites, but one must point out the many challenges that exist in achieving what we might call ‘quantitative delivery’ of a drug. By and large such efforts have led to 5–10% accumulation of nanoparticles in tumour sites, which translates to a 5–10% drug load only when the nanoparticles are loaded to the highest level. This is a formulation challenge involving the compatibility of drugs, macromolecules and proteins with the materials that make up the nanosystems.
The challenge also partly rests in the changing physical and genetic nature of tumours as they grow and the biological complexities of other targets. That is, the target is a moving target. We will consider some of the factors that fall within the scope of this text.
14.7.1 Complexity of particle flow9
Drug targeting using nanoparticulate carriers depends on many factors to achieve quantitative accumulation in target sites. Particles must translocate from the site of injection to distant organs. To an extent, therefore, this depends on the flow patterns of nanoparticles in the blood and in interstitial spaces. The theoretical advantage of nanosystems is that their small size allows freer movement than that of, say, microspheres in the circulation, lymph and tissues. Flow rates are important also in the determination of the possibility and success of interaction of nanoparticles with endothelial receptors prior to internalisation. What is the influence, for example, of nanoparticle size on particle flow in the circulation? What is the influence of shape on flow and fate? Nanoparticles in vivo are driven by the blood flow to distant sites. Particle velocities can be size-dependent because the flow rates across the diameter of the vessels are not constant and hence particle segregation in polydisperse systems might occur, as shown in Fig. 14.20. Flow results in shearing forces at the point of interaction between nanoparticles and the target receptors; smaller particles suffer lower forces likely to dislodge them from receptor surfaces. This is illustrated in Fig. 14.21. Any aggregation of particles, which might even be flow-induced, will alter flow patterns and interactions with receptors.
Figure 14.20 Diagram of the velocity pattern in a tube of flowing liquid showing particles of different sizes separating according to their diameter. The large particles, being unable to approach close to the capillary wall, experience the faster fluid streamlines towards the axis and hence move more rapidly.
Figure 14.21 Processes occurring in the deposition of nanoparticles in flow conditions as a function of the range of interaction of forces (nanometres) and adhesion times. At the start, mass transport to the surface occurs, initial adhesion occurring through electrostatic attractions and van der Waals forces. Hydrophobic interactions can play their part as well as specific receptor–ligand interactions, which are short-range interactions. Physical adsorption of ligands to the surface of nanoparticles or covalent attachment of ligands changes the nature of the primary surface and may influence each of the forces referred to here.
Drawn with kind permission from Springer Science and Business Media after Vacheethanasee K, Marchant RE. Non-specific Staphylococcus epidermidis adhesion: contributions of bacterial hydrophobicity and charge. In: An YH, Friedman RJ (eds) Handbook of Bacterial Adhesion: Principles, Methods and Applications. Totowa, NJ: Humana Press; 2000: 73–90.
Larger particles close to the walls of the vessels experience greater shearing forces because of the nature of the flow patterns shown. Adhesion, seen as a prerequisite to cellular uptake from blood and interstitial fluids, is not a foregone conclusion. The determining factors include particle diameter, flow rate, the density of receptors and the force of attraction between particle and receptor.
Figure 14.21 simply reinforces previous points and considers in greater detail the forces involved in nanoparticle interaction with biological surfaces. Particles have to be close to the surface to experience the force of attraction, which allows initial contact with membranes. This diagram again should clearly demonstrate the importance of physical phenomena in the biological environment.
14.7.2 Aggregation of particles
Given their large surface to volume/weight ratios, nanoparticles are prone to aggregate. The formulation of a stable nanoparticle suspension in the laboratory is one thing; the maintenance of the monodisperse state in vivo is another. Particles will have moved from an aqueous medium (of the formulation) to a more complex situation in blood or tissues. There are biological consequences because opsonins adsorb from the plasma on to the surface of the nanoparticles, which leads to uptake by the reticuloendothelial system. (An opsonin is any molecule that acts as a binding enhancer for the process of phagocytosis, for example, by coating the surface of injected particles and other foreign objects.) In terms of the pharmaceutics of the nanosystems, the potential of the particles to aggregate is critical. Aggregation changes the hydrodynamic size of the particles, decreases their diffusion, restricts extravasation and reduces the effective surface area for interactions with receptors.
PEGylated nanoparticles
PEGylation involves the covalent attachment of polyoxyethylene glycol (PEG) chains to the surface of the particles. PEGylation (Fig. 14.22) of course creates a hydrated surface on hydrophobic particles, which allows for both steric and enthalpic stabilisation. It also reduces opsonin adsorption, leading to increased circulation times and the enhanced statistical possibility of nanoparticle uptake after multiple passages through the circulation. PEGylation changes the nature of the surface, turning hydrophobic surfaces into hydrophilic ones. The effect of PEG depends on its chain length.10 PEG 5000 has a greater effect than PEG 2000 or PEG 750, as predicted by calculations of enthalpic and entropic stabilisation (see Chapter 6).
Figure 14.22 A representation of (a) a particle PEGylated with a polyoxyethylene-based block copolymer and (b) a block copolymer micelle with the external hydrophilic chains. The hydrophilic polymer chains on adjacent particles provide both a physical and thermodynamic barrier to aggregation. See also Chapter 6.
14.7.3 Nanoparticle diffusion
The Stokes–Einstein equation (see Chapter 2) relates the diffusion coefficient, D, of a molecule or object to the continuous-phase viscosity η and the radius of the diffusing particle, r, as was discussed earlier in the chapter, in the form D = kT/6πηr.
In any one liquid medium, e.g. water, the diffusion coefficient of spherical particles is inversely related to their radius. This alone is important in thinking of the translocation of nanoparticles towards their targets in tissues. Diffusion is important in free solution, in cell culture media, in the gel-like interior of cells, even perhaps in the cell nucleus and in the extracellular matrix. In the brain, for example, diffusion of drugs from nanoparticles or implants is slow and the radius of spread of a drug like paclitaxel after release from implants or nanoparticles is of the order of only millimetres. Gels have been used as models for diffusion of nanoparticles in complex media: the Stokes–Einstein equation applies and the ratio of diffusion in the gel (D) to diffusion in water (D0) can be calculated. In gels the viscosity in the equation is not the bulk viscosity but the so-called microscopic viscosity,11 η0, which is actually close to that of water, as it is the viscosity of the medium in which nanoparticles move between the entangled chains of the macromolecular gels. When the diameter of the particles reaches a critical size in gels, a size approaching the nominal diameter of the pores, diffusion asymptotically approaches zero.
Diffusion within cells is even more complex than within simple gels: first, the cytoplasm is a molecularly crowded zone, a complex gel with structural obstacles such as actin and myosin fibrils and strands. There is the additional tortuosity that occurs in gels as the moving particle avoids the regions of the macromolecular chains and the obstruction effects from the impenetrable regions of the cytoplasm. If we designate by De the effective diffusion coefficient and by D0 the coefficient in water we can write, where τ is the tortuosity factor and ε is the void fraction available to the diffusing solute:
ε = (1 − ) where
is the volume fraction of the polymer or other materials that are in the path of the diffusing material. DG (the diffusion coefficient in the gel) can be written as a ratio with the coefficient in water12:
If = 0.5, DG is 0.111 times that in water. When
= 0.8, DG
0.012D0. The binding of particles to actin reduces the number of free particles and hence further reduces flux.
Nanoparticle size is important in diffusion in complex media because the diffusional space may be restricted when the diameter of the particle exceeds the ‘pore’ diameter. The diffusion coefficients of 6 nm dendrimers in the cytoplasm of Caco2 cells and SK/MES-1 cells13 were determined to be 9.8 × 10−11 cm2 s−1 and 6 × 10−11 cm2 s–1, respectively, some 1000 times lower than that in water. Adsorption, obstruction and entrapment occur, and at a certain particle radius diffusion virtually ceases.
There are other issues, as shown in Fig. 14.23, where particles of different sizes passing through a gel can be filtered according to their diameters, or two different types of particles can be filtered according to their adhesivity towards the fibres of the gel (or, for example, actin fibres in cells).
Figure 14.23 Diagrammatic representation of a hydrogel such as mucus: (left) particle filtering by size, the larger particles being held back, and (right) interaction filtering of two particle types, by interaction of one type with the gel strands.
Reproduced from Lieleg O et al. Characterization of particle translocation through mucin hydrogels. Biophys J 2010;98:1782–1789. Courtesy of Elsevier 2010.
14.7.4 Stochastic processes
There can be a tendency to think that events such as ligand–receptor interactions, diffusion of molecules or nanoparticles from A to B, or nanoparticle uptake by specific cells or organs are inevitable. Given time all things might happen. One of the principles of ensuring the long circulation of particles is to enhance their chances of extravasation and interaction with appropriate surfaces. But the movement of molecules free in solution is the result of a number of random walks. This randomness causes events to be referred to as stochastic. The chance of an individual nanoparticle extravasating on its passage along a capillary, the chance of a nanoparticle ligand adhering to the appropriate receptor, and so on. Figure 14.24 illustrates Brownian motion of a nanoparticle, motion of particle in confined spaces and a cartoon of the effect of random walks on cell uptake.
Figure 14.24 Random walks of nanoparticles (top left) of particles in Brownian motion and (top right) in a confined space (such as a cell or large liposome) (Al-Obaidi and Florence). Lower cartoon: the complex track before cell uptake.
|
Physicochemical properties are important in determining the biological fate of nanoparticles:
|
14.8 Detoxification: reducing blood levels
The ingenious converse of drug delivery with carriers such as liposomes is to administer empty carriers which will absorb excess drug in the circulation as a means of detoxification, as demonstrated by Leroux and his colleagues (Fig. 14.25).
Figure 14.25 Representation of the reduction of plasma levels of a drug above its toxic levels by the administration of empty liposomes. The carrier may contain enzymes to metabolise the absorbed drug where appropriate. MTL, minimum toxic level.
Reproduced by permission from Macmillan Publishers Ltd from Leroux JC et al. Injectable nanocarriers for biodetoxification. Nature Nanotech 2007;2:679–684. Copyright 2007.
14.9 Regulatory challenges: characterisation
Regulatory challenges generally have evolved for good reasons to ensure reproducibility and quality in vitro and in vivo. Parameters that must be closely defined for nanosystems include those in Table 14.3.
Table 14.3 Parameters and questions that must be addressed before clinical use of nanosystems
N.B.: Targeted systems congregate in specific organs, and hence can alter the toxicological balance. |
Summary
This chapter covers some of the physicochemical principles of the preparation and the use of nanoparticles as drug delivery vectors. It should be clear from this chapter that physical and chemical properties of nanoparticles are crucial in determining their biological fate and success. In targeting it should be remembered that even decorated particles with specific ligands may not reach the site of action because transport to most sites of action and interactions between ligands and receptors are stochastic processes with many complications and barriers in the way. Nonetheless, continuing research will ensure that nanosystems will play a large part in the future of pharmaceuticals – as therapeutic carriers, as carriers of imaging agents or as excipients that are more precise than those that exist today.
References
1. Kreuter J. Nanoparticles and nanocapsules – new dosage forms in the nanometer size range. Pharm Acta Helv 1978;533:3–29.
2. Couvreur P et al. Nanocapsules: a new type of lysosomotropic carrier. FEBS Lett 1977;84:323–326.
3. Kreuter J. Nanoparticles – a historical perspective. Int J Pharm 2007;331:1–10.
4. Schulman JH et al. Mechanism of formation and structure of microemulsions by electron microscopy. J Phys Chem 1959;63:1677–1680.
5. Teeguarden JG et al. Particokinetics in vitro: dosimetry considerations for in vitro nanoparticle toxicity assessments. Toxicol Sci 2007;95:300–312.
6. Ambrosch A et al. LDL size distribution in relation to insulin sensitivity and lipoprotein patterning in young and healthy subjects. Diabetes Care 1998;21:2077–2084.
7. De TK et al. Polycarboxylic acid nanoparticles for ophthalmic drug delivery: an ex vivo evaluation with human cornea. J Microencapul 2004;21:841–855.
8. Pi Z et al. Preparation of ultrafine particles of azithromycin by sonochemical method. Nanomedicine 2007;3:86–88.
9. Florence AT. Nanoparticle flow: implications for drug delivery. In: Torchilin VP (ed.) Nanoparticulates as Drug Carriers. London: Imperial College Press; 2006: 9–27.
10. Mori A et al. Influence of the steric barrier of amphipathic poly(ethyleneglycol) and ganglioside GM1 on the circulation time of liposomes and on the target binding of immunoliposomes in vivo. FEBS Lett 1991;284:263–266.
11. Ruenraroengsak P, Florence AT. The diffusion of latex nanospheres and the effective (microscopic) viscosity of HPMC gels. Int J Pharm 2005;298:361–366.
12. Mackie JS, Meares P. The diffusion of electrolytes in a cationic membrane. I. Theoretical. Proc Roy Soc Lond A Math Phys Sci 1955;232:498–509.
13. Ruenraroengsak P et al. Cell uptake, cytoplasmic diffusion and nuclear access of a 6.5 nm diameter dendrimer. Int J Pharm 2007;331:215–219.
Further reading
Florence AT. ‘Targeting’ nanoparticles. The constraints of physical laws and physical barriers. J Control Rel 2012;164:115–124.
Crommelin DJA, Florence AT. Towards more effective advanced drug delivery systems. Int J Pharm 2013;454:496–511.
Date AA, Patravale VB. Current strategies for engineering drug nanoparticles. Curr Opinion Colloid Interface Sci 2004;9:222–235.
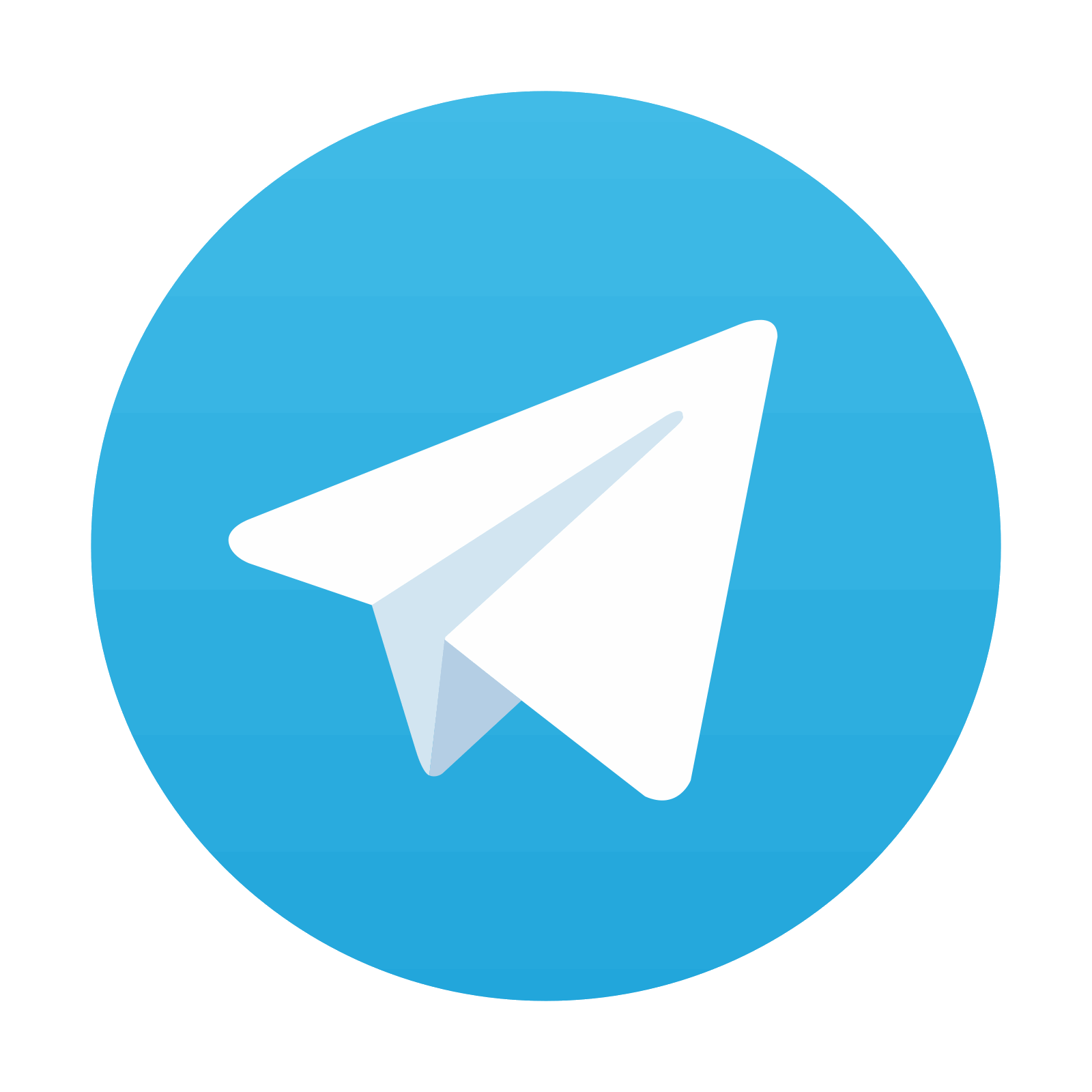
Stay updated, free articles. Join our Telegram channel
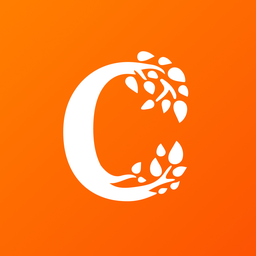
Full access? Get Clinical Tree
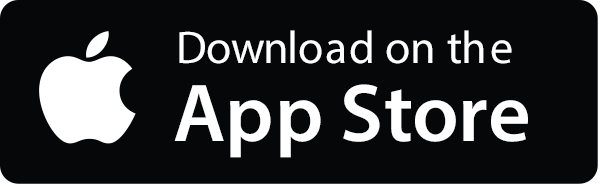
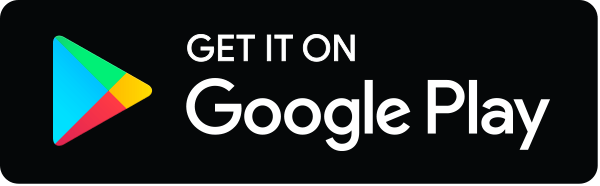