Virology: From Contagium Fluidum to Virome
Lynn W. Enquist
Vincent R. Racaniello
Virology has had a remarkable history. Even though humans did not realize viruses existed until the late 1880s, viral diseases have shaped the history and evolution of life on the planet. As far as we know, all living organisms, when studied carefully, are infected by viruses. These smallest microbes exert significant forces on every living thing, including themselves. The consequences of viral infections have not only altered human history, they have powerful effects on the entire ecosystem. As a result, virologists have gone to extraordinary lengths to study, understand, and eradicate these agents. It is noteworthy that just as the initial discovery of viruses required new technology (porcelain filters), uncovering the amazing biology underlying viral infections has gone hand in hand with new technology developments. Indeed, virologists have elucidated new principles of life processes and have been leaders in promoting new directions in science. For example, many of the concepts and tools of molecular biology and cell biology have been derived from the study of viruses and their host cells. This chapter is an attempt to review selected portions of this history as it relates to the development of new concepts in virology.
The Concept of Viruses as Infectious Agents
A diverse microbial world of bacteria, fungi, and protozoa had been widely accepted by the last half of the 19th century. An early proponent of the germ theory of disease was the noted German anatomist Jacob Henle of Gottingen (the discoverer of Henle’s loop and the grandfather of 20th-century virologist Werner Henle). He hypothesized in 1840 that specific diseases were caused by infectious agents that were too small to be observed with the light microscope. However, he had no evidence for such entities, and consequently his ideas were not generally accepted. It would take the work of Louis Pasteur and Henle’s student, Robert Koch, before it became evident that microbes could cause diseases.
Three major advances in microbiology came together to set the stage for the development of the concept of a submicroscopic agent that would come to be called a virus (e-Table 1.1). The first advance concerned spontaneous generation of organisms, which for years had been both supported and refuted by a variety of experiments. Louis Pasteur (1822–1895) used his swan-neck flasks to strike a mortal blow to the concept of spontaneous generation. Afterward Pasteur went on to study fermentation by different microbial agents. From his work he concluded that “different kinds of microbes are associated with different kinds of fermentations,” and he soon extended this concept to diseases. Pasteur’s reasoning strongly influenced Robert Koch (1843–1910), a student of Jacob Henle and a country doctor in a small German village. Koch developed solid media to isolate colonies of bacteria to produce pure cultures, and stains to visualize the microorganisms. With these tools in hand, Koch identified the bacterium that causes anthrax (Bacillus anthracis, 1876) and tuberculosis (Mycobacterium tuberculosis, 1882). Joseph Lister (1827–1912), a professor of surgery in Glasgow, had heard about Pasteur’s work, and he surmised that a sterile field should be maintained during surgery. Although many other scientists of that day contributed tools and concepts, it was principally Pasteur, Lister, and Koch who put together a new experimental approach for medical science.
These observations led Robert Koch to formalize some of Jacob Henle’s original ideas for defining whether a microorganism is the causative agent of a disease. Koch’s postulates state that (a) the organism must be regularly found in the lesions of the disease, (b) the organism must be isolated in pure culture, (c) inoculation of such a pure culture of organisms into a host should initiate the disease, and (d) the organism must be recovered once again from the lesions of the host. By the end of the 19th century, these concepts outlined an experimental method that became the dominant paradigm of medical microbiology. It was only when these rules broke down and failed to yield a causative agent that the concept of a virus was born.
The Birth of Virology
Pathogen Discovery, 1886–1903 (e-Table 1.1)
Adolf Mayer (1843–1942) was a German agricultural chemist and director of the Agricultural Experiment Station at Wageningen in The Netherlands when he was asked to investigate a disease of tobacco. He named the affliction tobacco mosaic disease after the dark and light spots that appeared on infected leaves (e-Fig. 1.1). To investigate the nature of the disease, Mayer inoculated healthy plants with the juice extracted from diseased plants by grinding up the infected leaves in water. Mayer reported that, “in nine cases out of ten (of inoculated plants), one will be successful in making the healthy plant … heavily diseased”.131 Although these studies established the infectious nature of the tobacco mosaic disease, neither a bacterial agent nor a fungal agent could be consistently cultured or detected in these extracts, so Koch’s postulates could not be satisfied. In a preliminary communication in 1882,130 Mayer speculated that the cause could be a “soluble, possibly enzyme-like contagium, although almost any analogy for such a supposition is failing in science.” Later Mayer concluded that the mosaic disease “is bacterial, but that the infectious forms have not yet been isolated, nor are their forms and mode of life known”.131
A few years later, Dimitri Ivanofsky (1864–1920), a Russian scientist working in St. Petersburg, was commissioned by the Russian Department of Agriculture to investigate the cause of a tobacco disease on plantations in Bessarabia, Ukraine, and the Crimea. Ivanofsky repeated Mayer’s observations by showing that the sap of infected plants contained an agent that could transmit the disease to healthy plants. But he added an important step—before the inoculation step, he passed the infected sap through a Chamberland filter (e-Fig. 1.2). This device, made of unglazed porcelain and perfected by Charles Chamberland, one of Pasteur’s collaborators, contained pores small enough to retard most bacteria. Ivanofsky reported to the Academy of Sciences of St. Petersburg on February 12, 1892, that “the sap of leaves infected with tobacco mosaic disease retains its infectious properties even after filtration through Chamberland filter candles”.94
Ivanofsky, like Mayer before him, failed to culture an organism from the filtered sap and could not satisfy Koch’s postulates. Consequently he suggested that a toxin (not a living, reproducing substance) might pass through the filter and cause the disease. As late as 1903, when Ivanofsky published his thesis,95 he still believed that he had been unable to culture the bacteria that caused this disease. Bound by the dogma of Koch’s postulates, Ivanofsky could not make a conceptual leap. It is therefore not surprising that Pasteur, who worked on the rabies vaccine145 at the same time (1885), never investigated the unique nature of the infectious agent.
The conceptual leap was provided by Martinus Beijerinck (1851–1931), a Dutch soil microbiologist who collaborated with Adolf Mayer at Wageningen. Unaware of Ivanofsky’s work, in 1898 Beijerinck independently found that the sap of infected tobacco plants could retain its infectivity after passage through a Chamberland filter. But he also showed that the filtered sap could be diluted and regain its “strength” after replication in living, growing tissue of the plant. This observation showed that the agent could reproduce (therefore, it was not a toxin) but only in living tissue, not in the cell-free sap of the plant. Suddenly it became clear why others could not culture the pathogen outside its host. Beijerinck called this agent a contagium vivum fluidum,10 or a contagious living liquid. He sparked a 25-year debate about whether these novel agents were liquids or particles. This conflict was resolved when d’Herelle developed the plaque assay in 191736 and when the first electron micrographs were taken of tobacco mosaic virus (TMV) in 1939.104
Mayer, Ivanofsky, and Beijerinck each contributed to the development of a new concept: a novel organism smaller than bacteria—an agent defined by the pore size of the Chamberland filter—that could not be seen in the light microscope, and could multiply only in living cells or tissue. The term virus, from the Latin for slimy liquid or poison,89 was at that time used interchangeably for any infectious agent, and so the agent of tobacco mosaic disease was called tobacco mosaic virus, or TMV. The literature of the first decades of the 20th century often referred to these infectious entities as filterable agents, and this was indeed the operational definition of viruses. Sometime later, the term virus became restricted in use to those agents that fulfilled the criteria developed by Mayer, Ivanofsky, and Beijerinck, and that were the first agents to cause a disease that could not be proven by using Koch’s postulates.
Shortly after this pioneering work on TMV, the first filterable agent from animals was identified by Loeffler and Frosch—foot-and-mouth disease virus.122 The first human virus discovered was yellow fever virus (1901), by Walter Reed and his team in Cuba.154
The years from 1930 to 1956 were replete with the discovery of a plethora of new viruses (e-Table 1.2). In fact, in this short time, virologists found most of the viruses we now know about. More fascinating perhaps is that these studies laid the groundwork for the birth of molecular virology.
Plant Viruses and the Chemical Period: 1929–1956
For the next 50 years, TMV played a central role in research that explored the nature and properties of viruses. With the development of techniques to purify proteins in the first decades of the 20th century came the appreciation that viruses were proteins and so could be purified in the same way. Working at the Boyce Thompson Institute in Philadelphia, Vinson and Petre (1927–1931) precipitated infectious TMV—using an infectivity assay developed by Holmes88—from the crude sap of infected plants using selected salts, acetone, or ethyl alcohol.193 They showed that the infectious virus could move in an electric field, just as proteins did. At the same time, H. A. Purdy-Beale, also at the Boyce Thompson Institute, produced antibodies in rabbits that were directed against TMV and could neutralize the infectivity of this agent.151 This observation was taken as further proof of the protein nature of viruses, although it was later realized that antibodies recognize chemicals other than proteins. With the advent of purification procedures for viruses, both physical and chemical measurements of the virus became possible. The strong flow birefringence of purified preparations of TMV was interpreted (correctly) to show an asymmetric particle or rod-shaped particle.180 Max Schlesinger,167 working on purified preparations of bacteriophages in Frankfurt, Germany, showed that the virions were composed of proteins and contained phosphorus and ribonucleic acid. This observation led to the first suggestion that viruses were composed of nucleoproteins. The crystallization of TMV in 1935 by Wendell Stanley,173 working at the Rockefeller Institute branch in Princeton, New Jersey, brought this infectious agent into the world of the chemists. Within a year, Bawden and Pirie8,9 had demonstrated that crystals of TMV contained 0.5% phosphorus and 5% RNA. The first “view” of a virus came from x-ray crystallography using these crystals to show rods of a constant diameter aligned in hexagonal arrays containing RNA and protein.16 The first electron micrographs of any virus were of TMV, and they confirmed that the virus particle is shaped like a rod105 (e-Fig. 1.3).
The x-ray diffraction patterns16 suggested that TMV was built from repeating subunits. These data and other considerations led Crick and Watson33 to realize that most simple viruses had to consist of one or a few species of identical protein subunits. By 1954–1955, techniques had been developed to dissociate TMV protein subunits, allowing reconstitution of infectious TMV from its RNA and protein subunits64 and leading to an understanding of the principles of virus self-assembly.25
The concept that viruses contained genetic information emerged as early as 1926, when H. H. McKinney reported the isolation of “variants” of TMV with a different plaque morphology that bred true and could be isolated from several geographic locations.132,133 Seven years later, Jensen confirmed McKinney’s observations101 and showed that the plaque morphology phenotype could revert. Avery’s DNA transformation experiments with pneumococcus5 and the Hershey-Chase experiment with bacteriophages,83 both demonstrated that DNA was genetic material. TMV had been shown to contain RNA, not DNA, and this nucleic acid was shown to be infectious, and therefore comprise the genetic material of the virus, in 195664,72—the first demonstration that RNA could be a genetic material. Studies on the nucleotide sequence of TMV RNA confirmed codon assignments for the genetic code, added clear evidence for the universality of the genetic code, and helped to elucidate the mechanisms of mutation by diverse agents.63 Research on TMV and related plant viruses has contributed significantly to both the origins of virology and its development as a science.
Bacteriophages
Early Years: 1915–1940
Frederick W. Twort was superintendent of the Brown Institution in London when he discovered viruses of bacteria in 1915. In his research, Twort was searching for variants of vaccinia virus (the smallpox vaccine virus), which would replicate in simple defined media outside living cells. In one of his experiments, he inoculated nutrient agar with an aliquot of the smallpox vaccine. The virus failed to replicate, but bacterial contaminants flourished on the agar medium. Twort noticed that some of these bacterial colonies changed visibly with time and became “watery looking” (i.e., more transparent). The bacteria within these colonies were apparently dead, as they could no longer form new colonies on fresh agar plates. He called this phenomenon glassy transformation. Simply adding the glassy transforming principle could rapidly kill a colony of bacteria. It readily passed through a porcelain filter, could be diluted a million-fold, and when placed upon fresh bacteria would regain its strength, or titer.188,189,190
Twort published these observations in a short note190 in which he suggested that a virus of bacteria could explain glassy transformation. He then went off to serve in World War I, and when he returned to London, he did not continue this research.
While Twort was puzzled by glassy transformation, Felix d’Herelle, a Canadian medical bacteriologist, was working at the Pasteur Institute in Paris. When a Shigella dysentery infection devastated a cavalry squadron of French soldiers just outside of Paris in August 1915, d’Herelle readily isolated and cultured the dysentery bacillus from filtered fecal emulsions. The bacteria multiplied and covered the surface of his agar plates, but occasionally d’Herelle observed clear circular spots devoid of growth. He called these areas taches vierges, or plaques. He followed the course of an infection in a single patient, noting when the bacteria were most plentiful and when the plaques appeared.35,36 Plaques appeared on the fourth day after infection and killed the bacteria in the culture dish, after which the patient’s condition began to improve.
d’Herelle found that a filterable agent, which he called a bacteriophage, was killing the Shigella bacillus. In the ensuing years he developed fundamental techniques in virology that are utilized to this day, such as the use of limiting dilutions to
determine the virus titer by plaque assay. He reasoned that the appearance of plaques showed that the virus was particulate, or “corpuscular,” and not a liquid as Beijerinck had insisted. d’Herelle also found that if virus was mixed with a host cell and then subjected to centrifugation, the virus was no longer present in the supernatant fluid. He interpreted this to mean that the first step of a virus infection is attachment, or adsorption, of virus to the host cell. Furthermore, viral attachment occurred only when bacteria sensitive to the virus were used, demonstrating that host specificity can be conferred at a very early step in infection. Lysis of cells and the release of infectious virus were also described in startlingly modern terms. d’Herelle clearly established many of the principles of modern virology.34,35
determine the virus titer by plaque assay. He reasoned that the appearance of plaques showed that the virus was particulate, or “corpuscular,” and not a liquid as Beijerinck had insisted. d’Herelle also found that if virus was mixed with a host cell and then subjected to centrifugation, the virus was no longer present in the supernatant fluid. He interpreted this to mean that the first step of a virus infection is attachment, or adsorption, of virus to the host cell. Furthermore, viral attachment occurred only when bacteria sensitive to the virus were used, demonstrating that host specificity can be conferred at a very early step in infection. Lysis of cells and the release of infectious virus were also described in startlingly modern terms. d’Herelle clearly established many of the principles of modern virology.34,35
Although d’Herelle’s bacteriophages lysed their host cells, by 1921 it had become apparent that under certain situations the virus and cell existed peacefully—a condition called lysogeny. In some experiments it became impossible to separate the virus from its host. This conundrum led Jules Bordet of the Pasteur Institute in Brussels to suggest that the transmissible agent described by d’Herelle was nothing more than a bacterial enzyme that stimulates its own production.22 Although incorrect, the hypothesis has remarkable similarities to modern ideas about prion structure and replication (see Chapter 77).
During the 1920s and 1930s, d’Herelle sought ways to use bacteriophages for medical applications, but he never succeeded. Furthermore, the basic research of the era was frequently dominated by the interpretations of scientists with the strongest personalities. Although it was clear that there were many diverse bacteriophages, and that some were lytic while some were lysogenic, their interrelationships remained ill defined. The highlight of this period was the demonstration by Max Schlesinger that purified phages had a maximum linear dimension of 0.1 micron and a mass of about 4 × 10−16 grams, and that they were composed of protein and DNA in roughly equal proportions.166,167 In 1936, no one quite knew what to make of that observation, but over the next 20 years it would begin to make a great deal of sense.
Phages and the Birth of Molecular Biology: 1938–1970 (e-Table 1.3)
Max Delbrück was trained as a physicist at the University of Göttingen, and his first position was at the Kaiser Wilhelm Institute for Chemistry in Berlin. There he joined a diverse group of individuals who were actively discussing how quantum physics related to an understanding of heredity. Delbrück’s interest in this area led him to develop a quantum mechanical model of the gene, and in 1937 he moved to the biology division at the California Institute of Technology to study genetics of Drosophila. Once there, he became interested in bacteria and their viruses, and teamed up with another research fellow, Emory Ellis,51 who was working with the T-even group of bacteriophages, T2, T4, and T6. Delbrück soon appreciated that these viruses were ideal for the study of virus replication, because they allowed analysis of how genetic information could determine the structure and function of an organism. Bacteriophages were also viewed as model systems for understanding cancer viruses or even for understanding how a sperm fertilizes an egg and a new organism develops. Together with Ellis, Delbrück showed that viruses reproduced in one step, in contrast to the multiplication of other organisms by binary fission.52 This conclusion was drawn from the elegant one-step growth curve experiment, in which an infected bacterium liberates hundreds of phages synchronously after a half-hour period during which viral infectivity was lost (e-Fig. 1.4). The one-step growth curve became the experimental paradigm of the phage group.
When World War II erupted, Delbrück remained in the United States (at Vanderbilt University) and met an Italian refugee, Salvador E. Luria, who had fled to America and was working at Columbia University in New York (on bacteriophages T1 and T2). After their encounter at a meeting in Philadelphia on December 28, 1940, they went to Luria’s laboratory at Columbia where they spent 48 hours doing experiments with bacteriophages. These two scientists eventually established the “phage group,” a community of researchers focused on using bacterial viruses as a model for understanding life processes. Luria and Delbrück were invited to spend the summer of 1941 at Cold Spring Harbor Laboratory, where they pursued research on phages. The result was that a German physicist and an Italian geneticist joined forces during the war years to travel throughout the United States and recruit a new generation of biologists (e-Fig. 1.5).
When Tom Anderson, an electron microscopist at the RCA Laboratories in Princeton, New Jersey, met Delbrück, the result was the first clear pictures of bacteriophages.126 At the same time, the first phage mutants were isolated and characterized.125 By 1946, the first phage course was being taught at Cold Spring Harbor, and in March 1947, the first phage meeting attracted eight people. From these humble beginnings grew the field of molecular biology, which focused on the bacterial host and its viruses.
Developing the Modern Concept of Virology (see e-Tables 1.3 to 1.5)
The next 25 years (1950–1975) was an intensely productive period of bacteriophage research. Hundreds of virologists produced thousands of publications that covered three major areas: (a) lytic infection of Escherichia coli with the T-even phages; (b) the nature of lysogeny, using lambda phage; and (c) the replication and properties of several unique phages such as φφX174 (single-stranded circular DNA), the RNA phages, and T7. This work set the foundations for modern molecular virology and biology.
The idea of examining, at the biochemical level, the events occurring in phage-infected cells during the latent period had come into its own by 1947–1948. Impetus for this work came from Seymour Cohen, who had trained first with Erwin Chargaff at Columbia University, studying lipids and nucleic acids, and then with Wendell Stanley working on TMV RNA. His research direction was established when after taking Delbrück’s 1946 phage course at Cold Spring Harbor, Cohen examined the effects of phage infection on DNA and RNA levels in infected cells using a colorimetric analysis. The results showed a dramatic alteration of macromolecular synthesis in infected cells. This included cessation of RNA accumulation, which later formed the basis for detecting a rapidly turning-over species of RNA and the first demonstration of messenger RNA (mRNA).4 DNA synthesis also halted, but for 7 minutes, followed by resumption at a 5- to 10-fold increased rate. At the same time, Monod and Wollman showed that the synthesis of a cellular enzyme, the inducible β-galactosidase, was inhibited
after phage infection.134 Based on these observations, the viral eclipse period was divided into an early phase, prior to DNA synthesis, and a late phase. More importantly, these results demonstrated that a virus could redirect cellular macromolecular synthetic processes in infected cells.32
after phage infection.134 Based on these observations, the viral eclipse period was divided into an early phase, prior to DNA synthesis, and a late phase. More importantly, these results demonstrated that a virus could redirect cellular macromolecular synthetic processes in infected cells.32
By the end of 1952, two experiments had a critical effect on virology. First, Hershey and Chase asked whether viral genetic information is DNA or protein. They differentially labeled viral proteins (35SO4) and nucleic acids (32PO4), and allowed the “tagged” particles to attach to bacteria. When they sheared the viral protein coats from the bacteria using a Waring blender, only DNA was associated with the infected cells.83 This result proved that DNA had all the information needed to reproduce new virus particles. A year later, the structure of DNA was elucidated by Watson and Crick, a discovery that permitted full appreciation of the Hershey-Chase experiment.195 The results of these two experiments formed a cornerstone of the molecular biology revolution.26
While these blockbuster experiments were being carried out, G. R. Wyatt and S. S. Cohen were quietly making another seminal finding.207 They identified a new base, hydroxymethylcytosine, in the DNA of T-even phages, which replaced cytosine. This began a 10-year study of how deoxyribonucleotides were synthesized in bacteria and phage-infected cells, and it led to the critical observation that the virus introduces genetic information for a new enzyme into the infected cell.60 By 1964, Mathews and colleagues had proved that hydroxymethylase does not exist in uninfected cells and must be encoded by the virus.32 These experiments introduced the concept of early enzymes, utilized in deoxypyrimidine biosynthesis and DNA replication,109 and provided biochemical proof that viruses encode new information that is expressed as proteins in an infected cell. At the same time, phage genetics became extremely sophisticated, allowing mapping of the genes encoding these viral proteins. Perhaps the best example of genetic fine structure was done by Seymour Benzer, who carried out a genetic analysis of the rII A and B cistrons of T-even phages with a resolution of a single nucleotide (without doing any DNA sequencing!).13 Studies on viral DNA synthesis, using phage mutants and cell extracts to complement and purify enzyme activities in vitro, contributed a great deal to our understanding of DNA replication.1 A detailed genetic analysis of phage assembly, utilizing the complementation of phage assembly mutants in vitro, revealed how complex structures are built by living organisms using the principles of self-assembly.47 The genetic and biochemical analysis of phage lysozyme helped to elucidate the molecular nature of mutations,176 and the isolation of phage amber mutations (nonsense mutations) provided a clear way to study second-site suppressor mutations at the molecular level.14 The circular genetic map of the T-even phages176 was explained by the circularly permuted, terminally redundant (giving rise to phage heterozygotes) conformation of these DNAs.186
The remarkable reprogramming of viral and cellular protein synthesis in phage-infected cells was dramatically revealed by an early use of sodium dodecyl sulfate (SDS)– polyacrylamide gels,112 showing that viral proteins are made in a specific sequence of events. The underlying mechanism of this temporal regulation led to the discovery of sigma factors modifying RNA polymerase and conferring gene specificity.75 The study of gene regulation at almost every level (transcription, RNA stability, protein synthesis, protein processing) was revealed from a set of original contributions derived from an analysis of phage infections.
Although this remarkable progress had begun with the lytic phages, no one knew quite what to make of the lysogenic phages. This situation changed in 1949 when André Lwoff began his studies with Bacillus megaterium and its lysogenic phages at the Pasteur Institute. By using a micromanipulator, Lwoff could show that single lysogenic bacteria divided up to 19 times without liberating a virus particle. No virions were detected when lysogenic bacteria were broken open by the investigator. But from time to time a lysogenic bacterium spontaneously lysed and produced many viruses.128 Ultraviolet light was found to induce the release of these viruses, a key observation that began to outline this curious relationship between a virus and its host.129 By 1954, Jacob and Wollman97,98 at the Pasteur Institute had made the important observation that a genetic cross between a lysogenic bacterial strain and a nonlysogenic recipient resulted in the induction of the virus after conjugation, a process they called zygotic induction. In fact, the position of the lysogenic phage or prophage in the chromosome of its host E. coli could be mapped by interrupting mating between two strains.98 This experiment was crucial for our understanding of lysogenic viruses, because it showed that a virus behaved like a bacterial gene on a chromosome in a bacterium. It was also one of the first experimental results to suggest that the viral genetic material was kept quiescent in bacteria by negative regulation, which was lost as the chromosome passed from the lysogenic donor bacteria to the nonlysogenic recipient host. This conclusion helped Jacob and Monod to realize as early as 1954 that the “induction of enzyme synthesis and of phage development are the expression of one and the same phenomenon”.128 These experiments laid the foundation for the operon model and the nature of coordinate gene regulation.
Although the structure of DNA was elucidated in 1953195 and zygotic induction was described in 1954, the relationship between the bacterial chromosome and the viral chromosome in lysogeny was still referred to as the attachment site and literally thought of in those terms. The close relationship between a virus and its host was appreciated only when Campbell proposed the model for lambda integration of DNA into the bacterial chromosome,27 based on the fact that the sequence of phage markers was different in the integrated state than in the replicative or vegetative state. This model led to the isolation of the negative regulator or repressor of lambda, a clear understanding of immunity in lysogens, and one of the early examples of how genes are regulated coordinately.150 The genetic analysis of the lambda bacteriophage life cycle is one of the great intellectual adventures in microbial genetics.82 It deserves to be reviewed in detail by all students of molecular virology and biology.
The lysogenic phages such as P22 of Salmonella typhimurium provided the first example of generalized transduction,210 whereas lambda provided the first example of specialized transduction.137 The finding that viruses could not only carry within them cellular genes, but transfer those genes from one cell to another, provided not only a method for fine genetic mapping but also a new concept in virology. As the genetic elements of bacteria were studied in more detail, it became clear that there was a remarkable continuum from lysogenic phages to episomes,
transposons and retrotransposons, insertion elements, retroviruses, hepadnaviruses, viroids, and prions. Genetic information moves between viruses and their hosts to the point where definitions and classifications begin to blur. The genetic and biochemical concepts that emerged from the study of bacteriophages made the next phase of virology possible. The lessons of the lytic and lysogenic phages were often relearned and modified as the animal viruses were studied.
transposons and retrotransposons, insertion elements, retroviruses, hepadnaviruses, viroids, and prions. Genetic information moves between viruses and their hosts to the point where definitions and classifications begin to blur. The genetic and biochemical concepts that emerged from the study of bacteriophages made the next phase of virology possible. The lessons of the lytic and lysogenic phages were often relearned and modified as the animal viruses were studied.
Animal Viruses
Cell Culture Technology and Discovery: 1898–1965 (see e-Tables 1.1 to 1.3)
Once the concept of viruses as filterable agents took hold, many diseased animal tissues were subjected to filtration to determine if a virus were involved. Filterable agents were found that were invisible in a light microscope, and replicated only in living animal tissue. There were some surprises, such as the transmission of yellow fever virus by a mosquito vector,154 specific visible pathologic inclusion bodies (virions and subviral particles) in infected tissue,95,142 and even viral agents that can “cause cancer”.50,159
Throughout this early time period (1900–1930), a wide variety of viruses were found (see e-Tables 1.1 and 1.2) and characterized with regard to their size (using the different pore sizes of filters), resistance to chemical or physical agents (e.g., alcohol, ether), and pathogenic effects. Based on these properties alone, it became clear that viruses were a very diverse group of agents. Some were even observable in the light microscope (vaccinia in dark-field optics). Some were inactivated by ether, whereas others were not. Viruses were identified that affected every tissue type. They could cause chronic or acute disease; they were persistent agents or recurred in a periodic fashion. Some viruses caused cellular destruction or induced cellular proliferation. For the early virologists, unable to see their agents in a light microscope and often confused by this great diversity, their studies certainly required an element of faith. In 1912, S. B. Wolbach, an American pathologist, remarked, “It is quite possible that when our knowledge of filterable viruses is more complete, our conception of living matter will change considerably, and that we shall cease to attempt to classify the filterable viruses as animal or plant”.204
The way out of this early confusion was led by the plant virologists and the development of techniques to purify viruses and characterize both the chemical and physical properties of these agents (see previous section, The Plant Viruses and the Chemical Period: 1929–1956). The second path out of this problem came from the studies with bacteriophages, where single cells infected with viruses in culture were much more amenable to experimental manipulation than were virus infections of whole animals. Whereas the plant virologists of that day were tethered to their greenhouses, and the animal virologists were bound to their animal facilities, the viruses of bacteria were studied in Petri dishes and test tubes. Nevertheless, progress was made in the study of animal viruses one step at a time: from studying animals in the wild, to laboratory animals, such as the mouse66 or the embryonated chicken eggs,205 to the culture of tissue, and then to single cells in culture. Between 1948 and 1955, a critical transition converting animal virology into a laboratory science came in four important steps: Sanford and colleagues at the National Institutes of Health (NIH) overcame the difficulty of culturing single cells163; George Gey at Johns Hopkins Medical School cultured and passaged human cells for the first time and developed a line of immortal cells (HeLa) from a cervical carcinoma71; and Harry Eagle at the NIH developed an optimal medium for the culture of single cells.46 In a demonstration of the utility of all these advanced, Enders and his colleagues showed that poliovirus could replicate in a nonneuronal human explant of embryonic tissues.54
These ideas, technical achievements, and experimental advances had two immediate effects on the field virology. They led to the development of the polio vaccine, the first ever produced in cell culture. From 1798 to 1949, all the vaccines in use (smallpox, rabies, yellow fever, influenza) had been grown in animals or embryonated chicken eggs. Poliovirus was grown in monkey kidney cells that were propagated in flasks.84,117 The exploitation of cell culture for the study of viruses began the modern era of molecular virology. The first plaque assay for an animal virus in culture was done with poliovirus,43 and it led to an analysis of poliovirus every bit as detailed and important as the contemporary work with bacteriophages. The simplest way to document this statement is for the reader to compare the first edition of General Virology by S. E. Luria in 1953124 to the second edition by Luria and J. E. Darnell in 1967,127 and to examine the experimental descriptions of poliovirus infection of cells. The modern era of virology had arrived, and it would continue to be full of surprises.
The Molecular and Cell Biology Era of Virology (see e-Tables 1.4 to 1.6)
The history of virology has so far been presented chronologically or according to separate virus groups (plant viruses, bacteriophages, animal viruses), which reflects the historical separation of these fields. In this section, the format changes as the motivation for studying viruses began to change. Virologists began to use viruses to probe questions central to understanding all life processes. Because viruses replicate in and are dependent on their host cells, they must use the rules, signals, and regulatory pathways of the host. By using viruses to probe cells, virologists began to make contributions to all facets of biology. This approach began with the phage group and was continued by the animal virologists. The recombinant DNA revolution also took place during this period (1970 to the present), and both bacteriophages and animal viruses played a critical and central role in this revolution. For these reasons, the organization of this section focuses on the advances in cellular and molecular biology made possible by experiments with viruses. Some of the landmarks in virology since 1970 are listed in e-Tables 1.4 to 1.6.
The Role of Animal Viruses in Understanding Eukaryotic Gene Regulation
The closed circular and superhelical nature of polyomavirus DNA was first elucidated by Dulbecco and Vogt42 and Weil and Vinograd.197 This unusual DNA structure was intimately related to the structure of the genome packaged in virions of simian vacuolating virus 40 (SV40). The viral DNA is wound around nucleosomes70; when the histones are removed, a superhelix is produced. The structure of polyoma viral DNA served as an excellent model for the E. coli genome206 and the mammalian
chromosome.113 Viral genomes have unique configurations not found in other organisms, such as single-stranded DNA (ssDNA),171 plus or minus strand RNA, or double-stranded RNA (dsRNA) as modes of information storage.
chromosome.113 Viral genomes have unique configurations not found in other organisms, such as single-stranded DNA (ssDNA),171 plus or minus strand RNA, or double-stranded RNA (dsRNA) as modes of information storage.
Many elements of the eukaryotic transcription machinery have been elucidated with viruses. The first transcriptional enhancer element (acts in an orientation- and distance-independent fashion) was described in the SV40 genome,76 as was a distance- and orientation-dependent promoter element observed with the same virus. The transcription factors that bind to the promoter, SP-1,44 or to the enhancer element, such as AP-1 and AP-2,116 and which are essential to promote transcription along with the basal factors, were first described with SV40. AP-1 is composed of fos and jun family member proteins, demonstrating the role of transcription factors as oncogenes.21 Indeed, the great majority of experimental data obtained for basal and accessory transcription factors come from in vitro transcription systems using the adenovirus major late promoter or the SV40 early enhancer–promoter.196 Our present-day understanding of RNA polymerase III promoter recognition comes, in part, from an analysis of the adenovirus VA gene transcribed by this polymerase.62
Almost everything we know about the steps of messenger RNA (mRNA) processing began with observations made with viruses. RNA splicing of new transcripts was first described in adenovirus-infected cells.15,31 Polyadenylation of mRNA was first observed with poxviruses,102 the first viruses shown to have a DNA-dependent RNA polymerase in the virion.103 The signal for polyadenylation in the mRNA was identified using SV40.59 The methylated cap structure found at the 5´ end of most mRNAs was first discovered on reovirus mRNAs.67 What little is known about the process of RNA transport out of the nucleus has shown a remarkable discrimination of viral and cellular mRNAs by the adenovirus E1B-55 Kd protein.147
Most of our understanding of translational regulation has come from studies of virus infected cells. Recruitment of ribosomes to mRNAs was shown to be directed by the 5´ cap structure first discovered on reovirus mRNAs. The nature of the protein complex that allows ribosomes to bind the 5´ cap was elucidated in poliovirus-infected cells, because viral infection leads to cleavage of one of the components, eIF4G. Internal initiation of translation was discovered in cells infected with picornaviruses (poliovirus and encephalomyocarditis virus).99,146 Interferon, discovered as a set of proteins that inhibits viral replication, was subsequently found to induce the synthesis of many antiviral gene products that act on translational regulatory events.92,93 Similarly, the viral defenses against interferon by the adenovirus VA RNA has provided unique insight into the role of eIF-2 phosphorylation events.108 Mechanisms for producing more than one protein from a eukaryotic mRNA (there is no “one mRNA one protein” rule in bacteria) were discovered in virus-infected cells, including polyprotein synthesis, ribosomal frameshifting, and leaky scanning. Posttranslational processing of proteins by proteases, carbohydrate addition to proteins in the Golgi apparatus, phosphorylation by a wide variety of important cellular protein kinases, or the addition of fatty acids to membrane-associated proteins have all been profitably studied using viruses. Indeed, a good deal of our present-day knowledge of how protein trafficking occurs and is regulated in cells comes from the use of virus-infected cell systems. The field of gene regulation has derived many of its central tenets from the study of viruses.
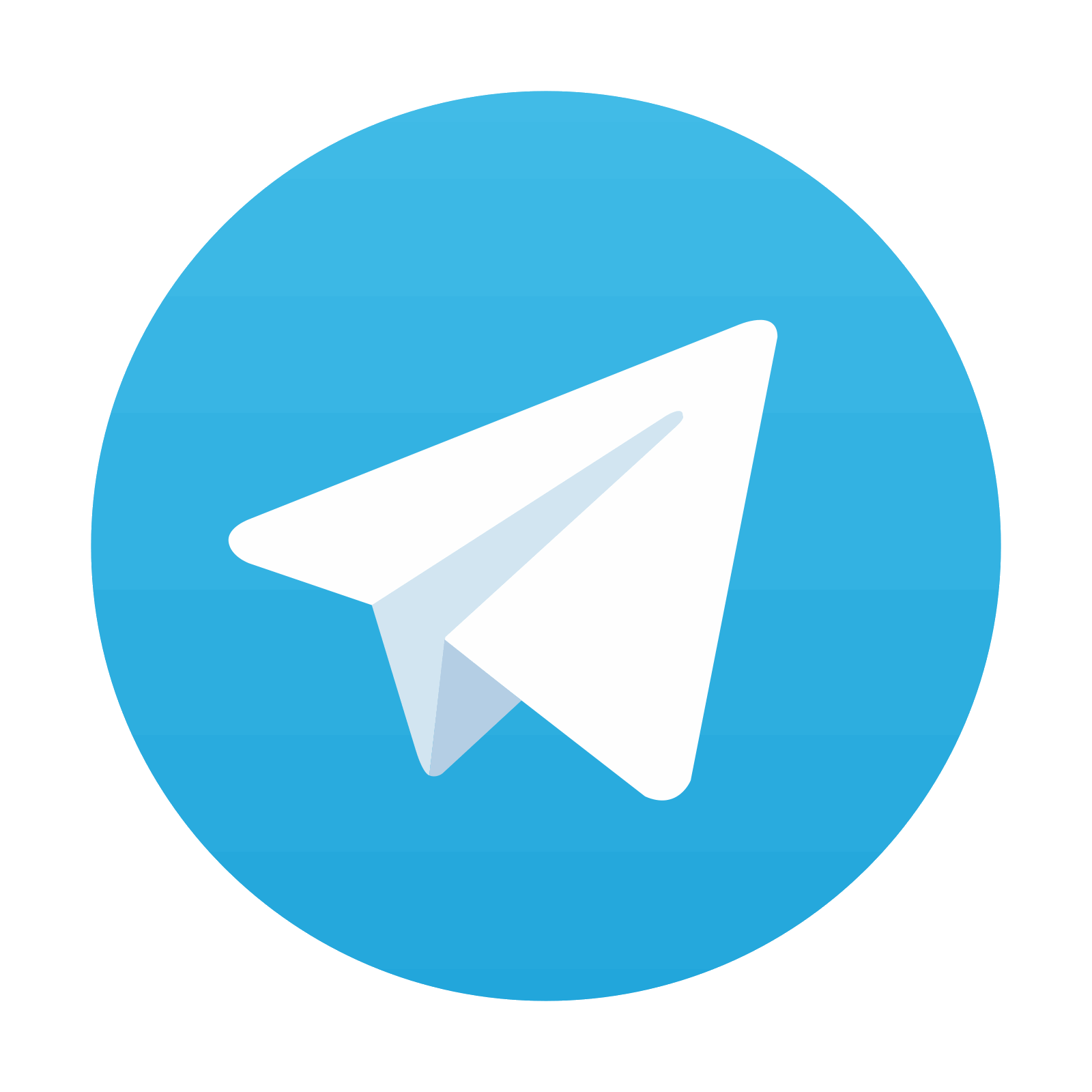
Stay updated, free articles. Join our Telegram channel
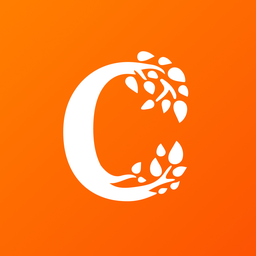
Full access? Get Clinical Tree
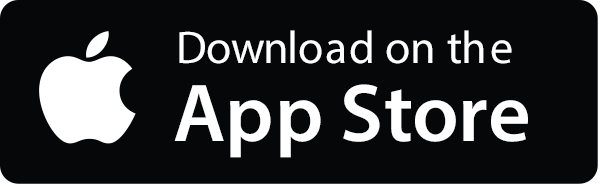
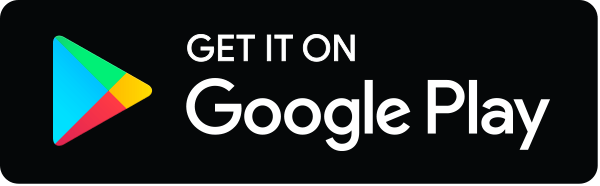