Fig. 8.1
Schematic explanation of α-, (α + β)-, and β-type titanium alloys based on crystal structure (Reprinted with permission from Ref. [10]. Copyright 2013, Jpn Soc For Bone Morphometry)
On the other hand, depending on the type of implant, a low Young’s modulus can sometimes prove detrimental [11]. For example, when applying a β-type titanium alloy, with a low Young’s modulus, to spinal fixation devices, the amount of springback in the device should ideally be as small as possible in order to improve its handling ability during operations [11]. This degree of springback is considered to be related to the Young’s modulus, with a lower Young’s modulus correlating to a larger springback. To put it more simply, a higher Young’s modulus is more desirable for surgeons, whereas a lower Young’s modulus is more desirable for patients. Consequently, in developing spinal fixation devices, it is necessary to satisfy the competing requirements of both the surgeon and the patient [11]. In order to address this issue, β-type titanium alloys with a partially changeable Young’s modulus have recently been developed. At the same time, removable β-type titanium alloys also have been developed [12], as removing implants is sometimes required, particularly in the case of younger people. As far as practical applications are concerned, the mechanical properties of biomaterials, such as fatigue properties, strength and elongation to fracture, fracture toughness, fretting fatigue properties, wear properties, and Young’s modulus, are all important factors. Among these, the fatigue strength (i.e., endurance) is particularly important. However, a means of improving fatigue strength without significantly increasing the Young’s modulus is desired.
In this chapter, the development of low Young’s modulus titanium alloys, self-adjustable Young’s modulus titanium alloys, and the enhancement of fatigue strength in titanium alloys with a low Young’s modulus are all described.
8.2 Development of Low Young’s Modulus Titanium Alloys
If the Young’s modulus of an implant is higher than that of the cortical bone, then the load is preferentially transferred to the implant. This phenomenon is referred to as stress shielding, and it is especially true in the case of load-bearing implants, such as those used for replacing failed hard tissue (cortical bone). When stress shielding occurs, it results in bone resorption and poor bone remodeling, which are the leading causes of implant loosening and refracture of the bone after implant extraction. As previously mentioned, the Young’s moduli of β-type titanium alloys are typically smaller than those of the other metallic biomaterials, such as stainless steels and Co–Cr alloys, as well as those of α- and (α + β)-type titanium alloys. For example, the Young’s moduli of SUS316L stainless steel, Co–Cr alloy, α-type pure titanium, and (α + β)-type Ti–6Al–4V ELI are 180, 210, 105, and 110 GPa, respectively [4]. Various low-modulus β-type titanium alloys have been developed for biomedical applications as shown in Table 8.1 [13]. Those low-modulus β-type titanium alloys which contain the most biocompatible elements are Ti–35Nb–7Zr–5Ta (TNZT: TiOsteum) [14], Ti–29Nb–13Ta–4.6Zr (TNTZ) [15], and Ti–36Nb–2Ta–3Zr–O (gum metal) [16]. Nb, Ta, and Zr are relatively safe elements for the human body based on reported cytotoxicity [17] [18] and metal allergy [19] data. Ti–35Nb–7Zr–5Ta (TNZT: TiOsteum) and Ti–29Nb–13Ta–4.6Zr (TNTZ), developed using a d-electron alloy design method based on the DV-Xα theory, and Ti–36Nb–2Ta–3Zr–O (gum metal: single crystal) exhibit Young’s modulus values of approximately 55, 60, and 70 GPa, respectively. Note, however, that these values are still much higher than that of cortical bone (10–30 GPa), and thus a means of lowering the Young’s modulus of the Ti–Nb–Ta–Zr β-type titanium alloy system is desirable.
Table 8.1
Representative low-modulus β-type titanium alloys for biomedical applications.
β-type titanium alloys | ASTM standard | ISO standard | JIS standard |
---|---|---|---|
Ti-13Nb-13Zr | ASTM F 1713 | – | – |
Ti- 12Mo-6Zr-2Fe (TMZF) | ASTM F 1813 | – | – |
Ti-12Mo-5Zr-5Sn | – | – | – |
Ti-15Mo | ASTM F 2066 | – | – |
Ti-16Nb-10Hf (Tiadyne 1610) | – | – | – |
Ti-15Mo-2.8Nb-0.2Si | – | – | – |
Ti-15Mo-5Zr-3Al | JIS T 7401-6 | ||
Ti-30Ta | – | – | – |
Ti-45Nb | AMS 4982 | – | – |
Ti-35Zr-10Nb | – | – | – |
Ti-35Nb-7Zr-5Ta (TNZT) | Task force F-04.12.23 | – | – |
Ti-29Nb- 13Ta-4.6Zr (TNTZ) | – | – | – |
Ti-35Nb-4Sn | – | – | – |
Ti-50Ta | – | – | – |
Ti-8Fe-8Ta | – | – | – |
Ti-8Fe-8Ta-4Zr | – | – | – |
Ti-35Nb-2Ta-3Zr | – | – | – |
Ti-22.5Nb-0.7Zr-2Ta | – | – | – |
Ti-23Nb-0.7Ta-2.0Zr- 1.2O (Gum Metal) | – | – | – |
Ti-28Nb-13Zr-0.5Fe (TNZF) | – | – | – |
Ti-24Nb-4Zr-7.9Sn (Ti2448) | – | – | – |
Ti-7.5Mo | – | – | – |
Ti-12Mo-3Nb | – | – | – |
Ti-12Mo-5Ta | – | – | – |
Ti-12Cr | – | – | – |
Ti-30Zr-7Mo | – | – | – |
Ti-30Zr-3Mo-3Cr | – | – | – |
Ti-5Fe-3Nb-3Zr | – | – | – |
Owing to the high cost of rare metal elements, such as Nb, Ta, Mo, and Zr, low-modulus β-type titanium alloys have recently been proposed that are based on lower-cost elements such as Fe, Cr, Mn, Sn, and Al. Examples of such alloys include Ti–10Cr–Al [20], Ti–Mn [21], Ti–Mn–Fe [22], Ti–Mn–Al [23], Ti–Cr–Al [24], Ti–Sn–Cr [25], Ti–Cr–Sn–Zr [26], Ti–(Cr, Mn)–Sn [27], and Ti–12Cr [11].
8.3 Reducing Young’s Modulus
The Young’s moduli of several β-type titanium alloys used for biomedical applications are typically around 80 GPa. However, the Young’s modulus of Ti–Nb–Ta–Zr alloys typically ranges between 55 and 70 GPa. Further reduction of the Young’s moduli of β-type titanium alloys, such as TNTZ, can be achieved by severe cold rolling. As shown in Fig. 8.2 [28], the Young’s modulus obtained with a high cold-working ratio is smaller than that without cold rolling. This decrease is attributable to the texture formation during cold rolling. A Young’s modulus of around 40 GPa has been achieved in Ti–35Nb–4Sn using a 90 % reduction, as shown in Fig. 8.3 [29]. Severe plastic deformation (SPD) is also effective in reducing the Young’s moduli of biomedical β-type titanium alloys. Figure 8.4 [30] shows the Young’s moduli of TNTZ subjected to severe cold rolling (TNTZCR) and the SPD process of high-pressure torsion (HPT) after 5, 10, 40, and 60 rotations, N. The Young’s modulus decreases as the texture increases, with an increase in N; a minimum Young’s modulus of around 60 GPa can be achieved at N = 60.
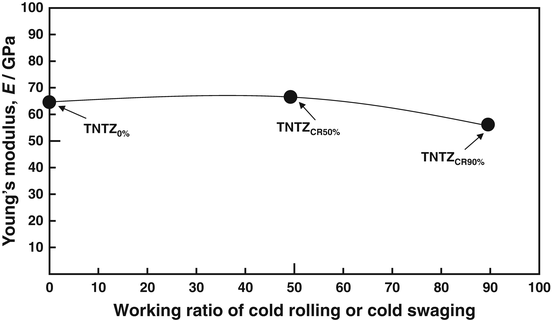
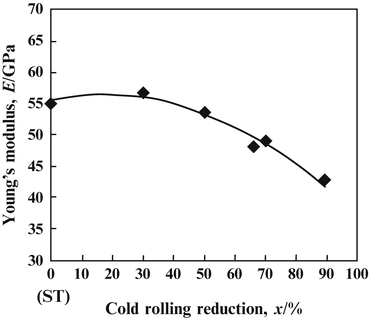
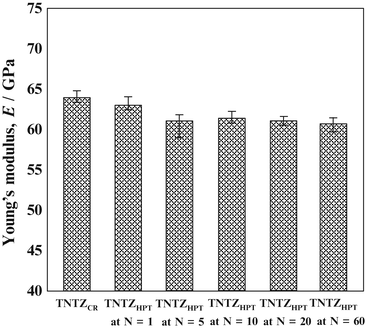
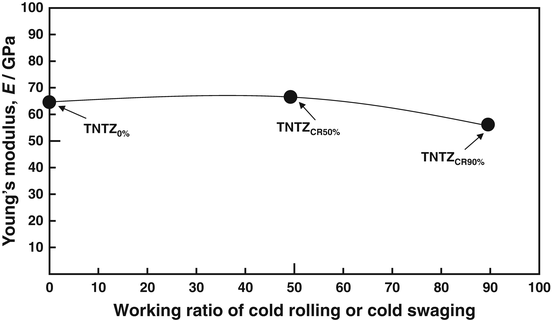
Fig. 8.2
Young’s modulus of TNTZ subjected to cold rolling as a function of working ratio (Reprinted with permission from Ref. [28]. Copyright 2010, Iron and Steel Inst. Jpn)
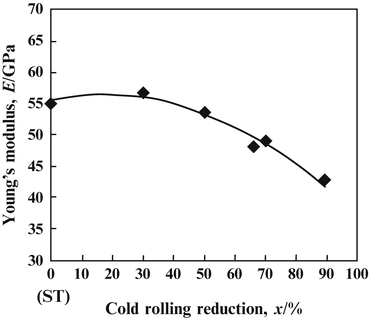
Fig. 8.3
Young’s modulus of Ti–35Nb–4Sn as a function of reduction. ST stands for solution treatment (Reprinted with permission from Ref. [29]. Copyright 2006, ASM Int)
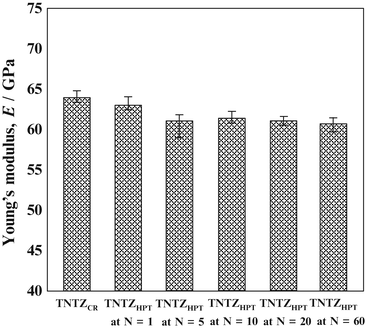
Fig. 8.4
Young’s moduli of TNTZCR and SPD TNTZHPT at N = 1–60. N stands for rotation number (Reprinted with permission from Ref. [30]. Copyright 2013, Elsevier B. V)
Since texture affects the Young’s moduli of polycrystalline β-type titanium alloys, a similar affect is expected for single crystals. The dependence of the Young’s modulus on the crystal direction was therefore investigated in monocrystalline TNTZ, the results of which are shown in Fig. 8.5 [31]. A Young’s modulus of around 35 GPa, which is comparable to the high-range Young’s modulus values of cortical bone, was measured in the <100> direction.
8.4 Titanium Alloys for Removable Implants
There are certain types of internal fixation devices that need to be removed after surgery in agreement with specific criteria. These include implants into the bone marrow, such as femoral, tibial, and humeral marrow, screws used for bone plate fixation [32], and some implants used for children, which would otherwise grow into the bone. Implant removal can result in undesirable results, such as palpable hardware, wound dehiscence, and/or exposure of the hardware [33, 34]. Any assimilation of the removable internal fixators with the bone, due to the precipitation of calcium phosphate, can cause refracture of the bone during the fixator-removal operation. In such cases, it is essential to prevent adhesion between the alloys and bone tissues. Thus, the precipitation of calcium phosphate must be inhibited.
Zirconium and titanium belong to the same group (i.e., same column in the periodic table), have the same crystal structure, and have unlimited solubility in each other [35]. Furthermore, zirconium is expected to be an effective element for solution strengthening and, with respect to toxicity, is no more harmful than titanium [36, 37]. Zirconium also has the ability to prevent the precipitation of calcium phosphate [32, 38], the main component in bones [39], and this holds true for titanium alloys with zirconium contents exceeding 25 mass%. Moreover, the tensile strength of Ti–Zr alloys is fairly high, although the elongation to failure is reduced when the zirconium content exceeds 56 mass% [40]. Overall, binary Ti–Zr alloys with high zirconium contents exhibit a combination of high strength [40–43] and excellent biocompatibility [37]. On the basis of these findings, biomedical Ti–Zr-based alloys such as Ti–Zr–Nb [44], Ti–Zr–Nb–Ta [45], and Ti–Zr–Al–V [44] have been recently developed. Additionally, Ti–30Zr–Mo [12] has also been developed in titanium alloys optimized for use in removable implants.
The Young’s moduli of Ti–30Zr–xMo alloys subjected to solution treatment are compared to other biomedical materials in Fig. 8.6 [12]. The Young’s moduli of Ti–30Zr–xMo alloys are lower than those of the other alloys considered with the exception of TNTZ. The addition of Mo to Ti–30Zr reduces the Young’s modulus, where a minimum value of around 60 GPa (a reduction of 34 % compared with Ti–30Zr) is achieved when 6Mo is added. TNTZ, Ti–30Zr–6Mo, and Ti–30Zr–7Mo exhibit the lowest Young’s modulus values of all those compared in Fig. 8.6. Figure 8.7 [12] compares the elastic admissible strain against elongation to failure for a distribution of as-solutionized Ti–30Zr–xMo alloys along with other biomedical materials. Typically in orthopedic applications, an ideal biomedical implant material is one which exhibits both a high strength and a low Young’s modulus. The elastic admissible strain, defined as the strength-to-modulus ratio, is therefore a useful parameter for consideration in such applications, a higher value indicating that the material is more suitable [46]. From Fig. 8.7, it can be seen that Ti–30Zr–6Mo and Ti–30Zr–7Mo exhibit a more attractive combination of higher elongation to failure and higher elastic admissible strain than those of the other Ti–30Zr–xMo alloys as well as the other materials considered for comparison, including SUS316L stainless steel, commercially pure titanium (CP Ti), Ti–6Al–4V ELI, and TNTZ. In addition, both Ti–30Zr–6Mo and Ti–30Zr–7Mo exhibit a lower Young’s modulus (almost three-fifths that of Ti–6Al–4V ELI), as shown in Fig. 8.6 [12]. These two alloys therefore show potential as candidates for use in biomedical applications.
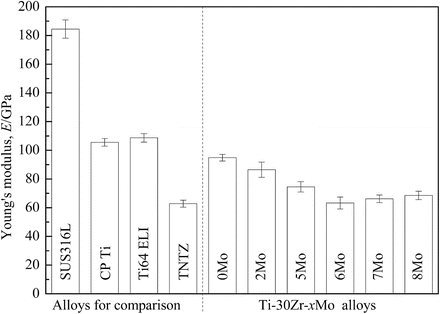
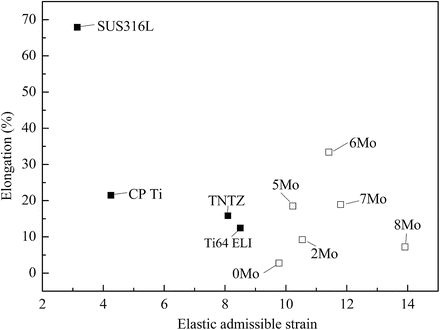
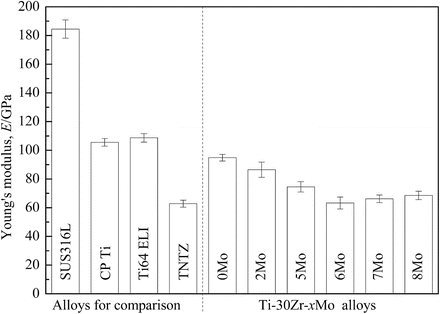
Fig. 8.6
Young’s moduli of Ti–30Zr–xMo (x = 0, 2, 5, 6, 7, and 8 wt.%) alloys subjected to solution treatment and SUS316L stainless steel (SUS316L), commercially pure titanium (CP Ti), Ti–6Al–4V ELI (Ti64 ELI), and TNTZ for comparison (Reprinted with permission from Ref. [12]. Copyright 2011, Elsevier B. V)
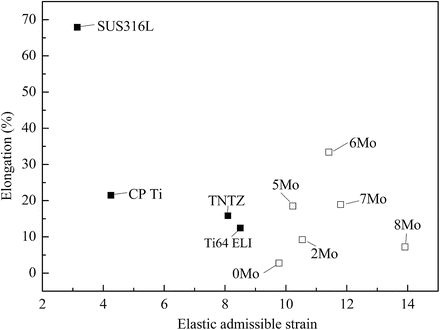
Fig. 8.7
A plot of elastic admissible strain versus elongation to fracture for a distribution of solutionized Ti–30Zr–xMo (x = 0, 2, 5, 6, 7, and 8 mass%) alloys and other biomedical materials (Reprinted with permission from Ref. [12]. Copyright 2011, Elsevier B. V)
Ti–30Zr–7Mo, on the basis of its low Young’s modulus, high elastic admissible strain, large elongation, and high 0.2 % proof stress, was selected for cytocompatibility testing [12]. Figure 8.8 [12] shows the density of cells cultured in Ti–30Zr–7Mo after 86.4 ks (24 h), which is considerably greater than that observed for the other materials compared. The formation of sulfite and/or sulfide in SUS316L stainless steel is believed to be the reason for the relatively low cell density measured for this alloy when compared with CP Ti, Ti–6Al–4V ELI, and TNTZ, all of which garnered similar cell density values.
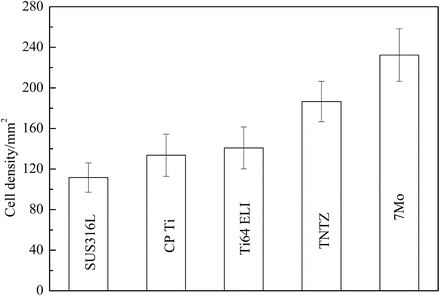
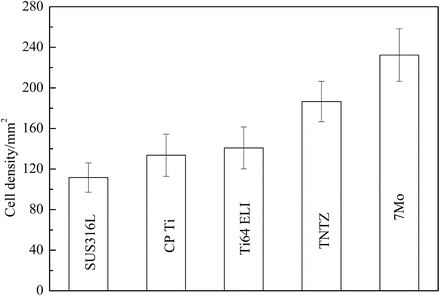
Fig. 8.8
A plot comparing the density of cells cultured in 24 h, during a cytocompatibility study, on samples of Ti–30Zr–7Mo and other biomedical materials (Reprinted with permission from Ref. [12]. Copyright 2011, Elsevier B. V)
8.5 Titanium Alloys with a Self-Tunable Young’s Modulus
For spinal fixation devices, which represent a specific type of orthopedic implant, a low Young’s modulus is preferred for the formation of healthy bones [47]. However, during spinal fixation operations, surgeons are required to bend such devices in order to reproduce the physiological curvature of the patient’s spine [48]. This bending sometimes has to be performed within a limited space inside the patient’s body. Therefore, a large springback is problematic for the formation of the desired shape of the devices. The degree of springback depends mainly on the strength and Young’s modulus; a lower Young’s modulus leads to a higher degree of springback. As a result, the Young’s modulus of titanium alloys for spinal fixation devices must be sufficiently low to avoid the stress shielding effect, yet high enough to suppress springback [49]. New types of titanium alloys, in which the Young’s moduli prior to deformation are low but increase during deformation (i.e., self-tuning Young’s moduli), have therefore been proposed. Of these alloys, Ti–Cr was the first to be developed, followed later by Ti–17Mo [50], Ti–30Zr–5Cr [51], Ti–30Zr–7Mo [52], and Ti–30Zr–3Mo–3Cr [51]. At the same time, Ti–30Zr–5Cr [51] and Ti–30Zr–3Mo–3Cr [51] were also developed for use as removable titanium alloys.
The Young’s moduli of a titanium alloy with a self-tunable Young’s modulus (Ti–12Cr) and those for TNTZ subjected to solution treatment and cold rolling are shown in Fig. 8.9 [11]. Note that the Young’s moduli of both Ti–12Cr and TNTZ subjected to solution treatment are similar (~62–64 GPa). During cold rolling, TNTZ does not exhibit a deformation-induced phase transformation, and therefore there is almost no change in its Young’s modulus. On the other hand, an increase in Young’s modulus due to cold rolling is observed in Ti–12Cr. The increase in Young’s modulus is a result of the deformation-induced ω-phase transformation, which occurs during cold rolling in Ti–12Cr as confirmed using a transmission electron microscopy [11]. The Young’s moduli of β-type titanium alloys are increased by the formation of the ω-phase [53], and this explains the increased Young’s modulus observed in cold-rolled Ti–12Cr [11, 54]. Figure 8.10 [11] shows the density of cells cultured in Ti–12Cr for 86.4 ks (24 h) in comparison with other alloys. Ti–12Cr exhibited the highest cell density.
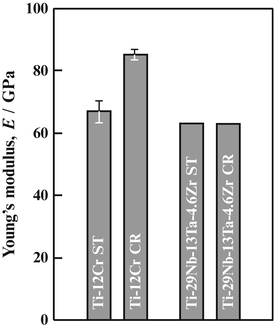
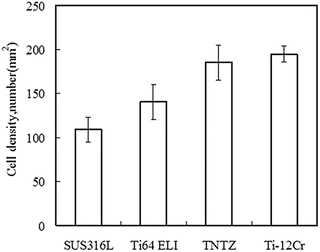
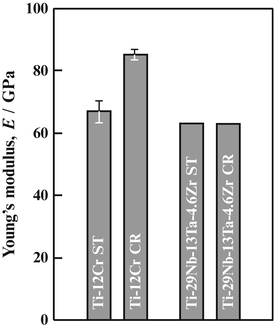
Fig. 8.9
Young’s moduli of Ti–12Cr and TNTZ subjected to solution treatment (ST) and cold rolling (CR) (Reprinted with permission from Ref. [11]. Copyright 2011, Elsevier B. V)
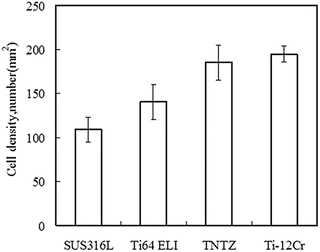
Fig. 8.10
Density of MC3T3-E1cells cultured in Ti–12Cr alloy subjected to solution treatment and SUS316L stainless steel (SUS316L), Ti–6Al–4V ELI (Ti64 ELI), and TNTZ considered for comparison for 86.4 ks (Reprinted with permission from Ref. [55]. Copyright 2012, Elsevier B. V)
Decreasing the chromium content in Ti–(10–12)Cr alloys can reduce the β(bcc)-lattice stability, as chromium acts as a β-stabilizing element. This, in turn, increases the amount of the ω-phase, which is separated into the athermal ω-phase, formed during quenching, and the deformation-induced ω-phase, caused by cold rolling. This would seem to suggest that a lower chromium content is beneficial for enhancing the ω-phase transformation in Ti–(10–12)Cr alloys. It is important to point out that the suppression of the athermal ω-phase transformation is likely to result in an increase in the deformation-induced ω-phase transformation [55]. Therefore, suppressing the formation of the athermal ω-phase in low-Cr Ti–Cr alloys should achieve not only a low Young’s modulus prior to deformation but also an increase in the deformation-induced ω-phase transformation after cold rolling, which would result in an increase in the Young’s modulus.
The athermal ω-phase in titanium alloys can be suppressed by oxygen addition [56, 57]. As a consequence, the addition of oxygen to Ti–Cr alloys increases the Young’s modulus of the alloy after deformation [58]. Figure 8.11 [58] shows the Young’s moduli of Ti–(11,12)Cr–(0.2, 0.4, 0.6)O alloys subjected to solution treatment (ST) and cold rolling (CR). For the alloys with identical chromium contents, the Young’s moduli increase with increasing oxygen content. This can be attributed to the solid solution-strengthening effect of oxygen as well as the aforementioned ω-phase transformation effect. Furthermore, the Young’s moduli of Ti–11Cr–0.2O and Ti–12Cr–0.2O are almost identical, whereas Ti–12Cr–0.4O and Ti–12Cr–0.6O have slightly higher Young’s moduli than those of Ti–11Cr–0.4O and Ti–11Cr–0.6O, respectively. The reasons for this have been reported as follows: For Ti–11Cr–0.2O and Ti–12Cr–0.2O, there is still a small amount of athermal ω-phase formed in the ST condition. Consequently, the Young’s moduli of these alloys are influenced by the competing effects of the athermal ω-phase, which can be suppressed by increasing the chromium content to stabilize the β-lattice, and the solid solution-strengthening effect of chromium. Conversely, because almost no athermal ω-phase forms in the Ti–(11,12)Cr–(0.4, 0.6)O alloys, the solid solution-strengthening effect of chromium becomes the primary reason for the change in Young’s modulus.
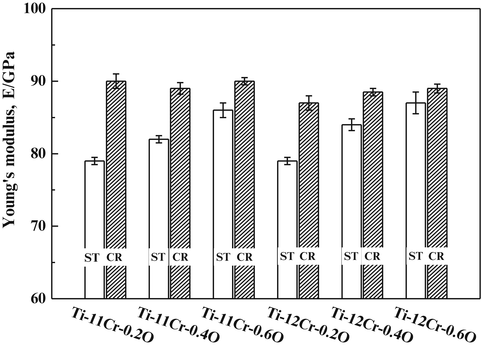
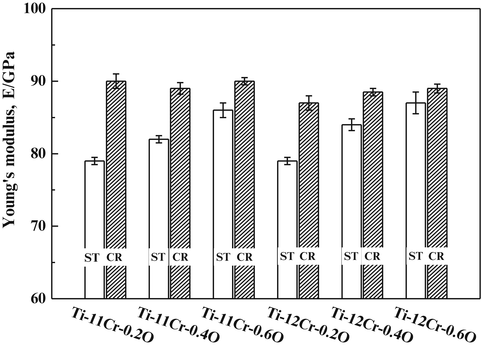
Fig. 8.11
Young’s moduli of Ti–(11,12)Cr–(0.2, 0.4, 0.6)O alloys subjected to ST and CR (Reprinted with permission from Ref. [58]. Copyright 2014, Elsevier B. V)
The Young’s modulus is always greater for the CR material compared to the ST material. Ti–11Cr–0.2O exhibits a Young’s modulus of less than 80 GPa in the ST condition, which is much lower than that for SUS316L stainless steel, CP Ti, and Ti–6Al–4V ELI. Ti–11Cr–0.2O also exhibits a Young’s modulus of more than 90 GPa in the CR condition. This change in Young’s modulus, which is the greatest change in Young’s modulus among all the materials examined, is desirable for satisfying the competing requirements of both surgeons and patients in spinal fixation applications. Figure 8.12 [58] depicts comparative profiles of the ratio of springback per unit stress as a function of the applied strain for TNTZ, Ti–30Zr–3Cr–3Mo, Ti–17Mo, Ti–12Cr, Ti–11Cr–0.2O, and Ti–6Al–4V ELI. These ratios all show a similar trend, with an initial significant decrease that then stabilizes with further increase in the applied strain. Ti–11Cr–0.2O reaches a low and stable ratio when the applied strain is greater than 2 %. It therefore exhibits a minimal ratio of springback per unit stress. The ratio for Ti–11Cr–0.2O is always much lower than that of TNTZ, and it is similar to that for Ti–6Al–4V ELI.
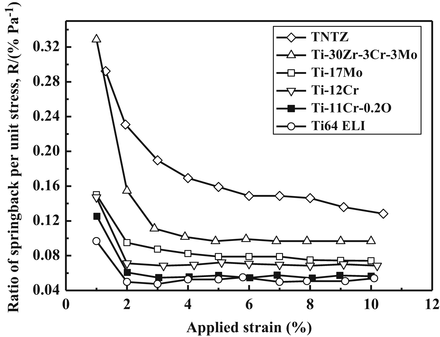
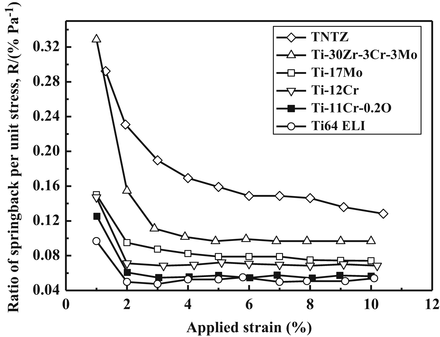
Fig. 8.12
Ratio of springback per unit stress (R) as a function of applied strain for Ti–11Cr–0.2O, TNTZ, Ti–30Zr–3Cr–3Mo, Ti–17Mo, Ti–12Cr, and Ti–6Al–4V ELI (Ti64 ELI) subjected to solution treatment (Reprinted with permission from Ref. [58]. Copyright 2014, Elsevier B. V)
To satisfy the requirements for spinal fixation applications, chromium can be added to TNTZ, which results in a higher deformation-induced Young’s modulus. Two newly designed alloys, TNTZ–8Ti–2Cr and TNTZ–16Ti–4Cr, possess a more stable β-phase than TNTZ, and their Young’s moduli increase in going from the ST condition to the CR condition, where a higher Young’s modulus value is exhibited by the alloy with the higher Cr content; see Fig. 8.13 [59]. These two alloys exhibit an increased Young’s modulus after cold rolling, with TNTZ–16Ti–4Cr exhibiting the greatest increase (~20 %, from ~64 GPa after solution treatment to ~77 GPa after cold rolling). These Cr-modified TNTZ alloys also show significantly less springback than the original TNTZ as demonstrated from the results of tensile and bending loading–unloading tests shown in Fig. 8.14 [59].
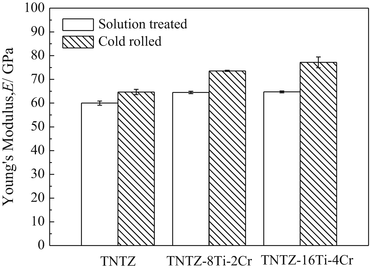
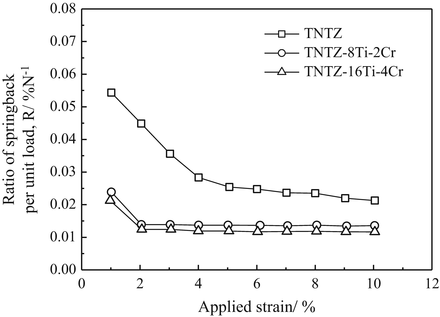
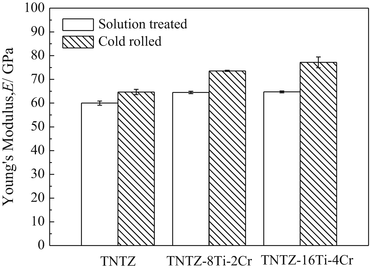
Fig. 8.13
Young’s modulus of TNTZ subjected to solution treatment and cold rolling and Cr-added TNTZ subjected to solution treatment and cold rolling (Reprinted with permission from Ref. [59]. Copyright 2013, Elsevier B. V)
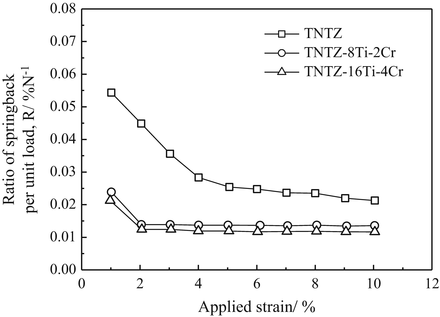
Fig. 8.14
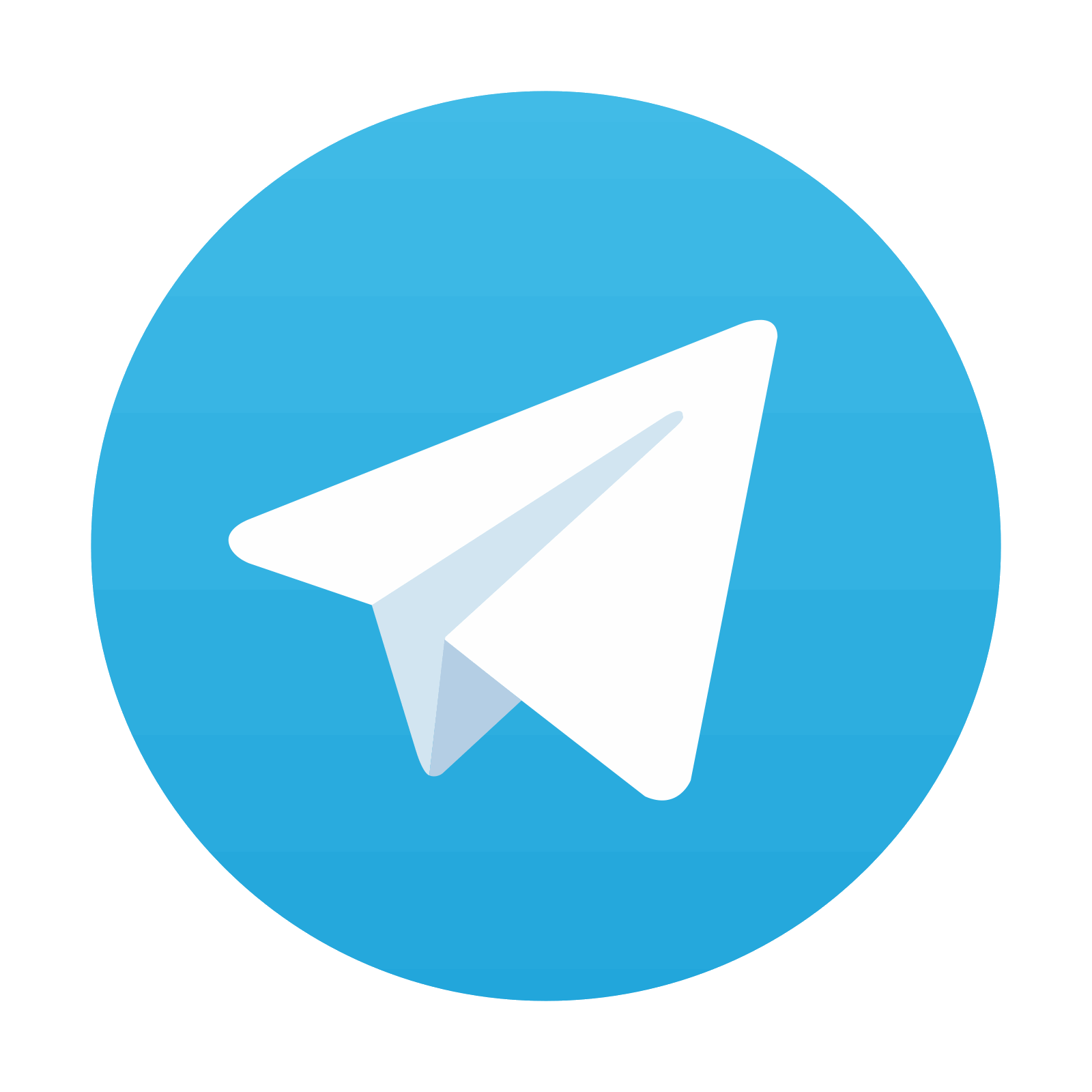
Ratio of springback per unit load (R) as a function of applied strain for TNTZ and Cr-added TNTZ subjected to solution treatment (TNTZ–ST, TNTZ–8Ti–2Cr–ST, and TNTZ–16Ti–4Cr–ST) (Reprinted with permission from Ref. [59]. Copyright 2013, Elsevier B. V)
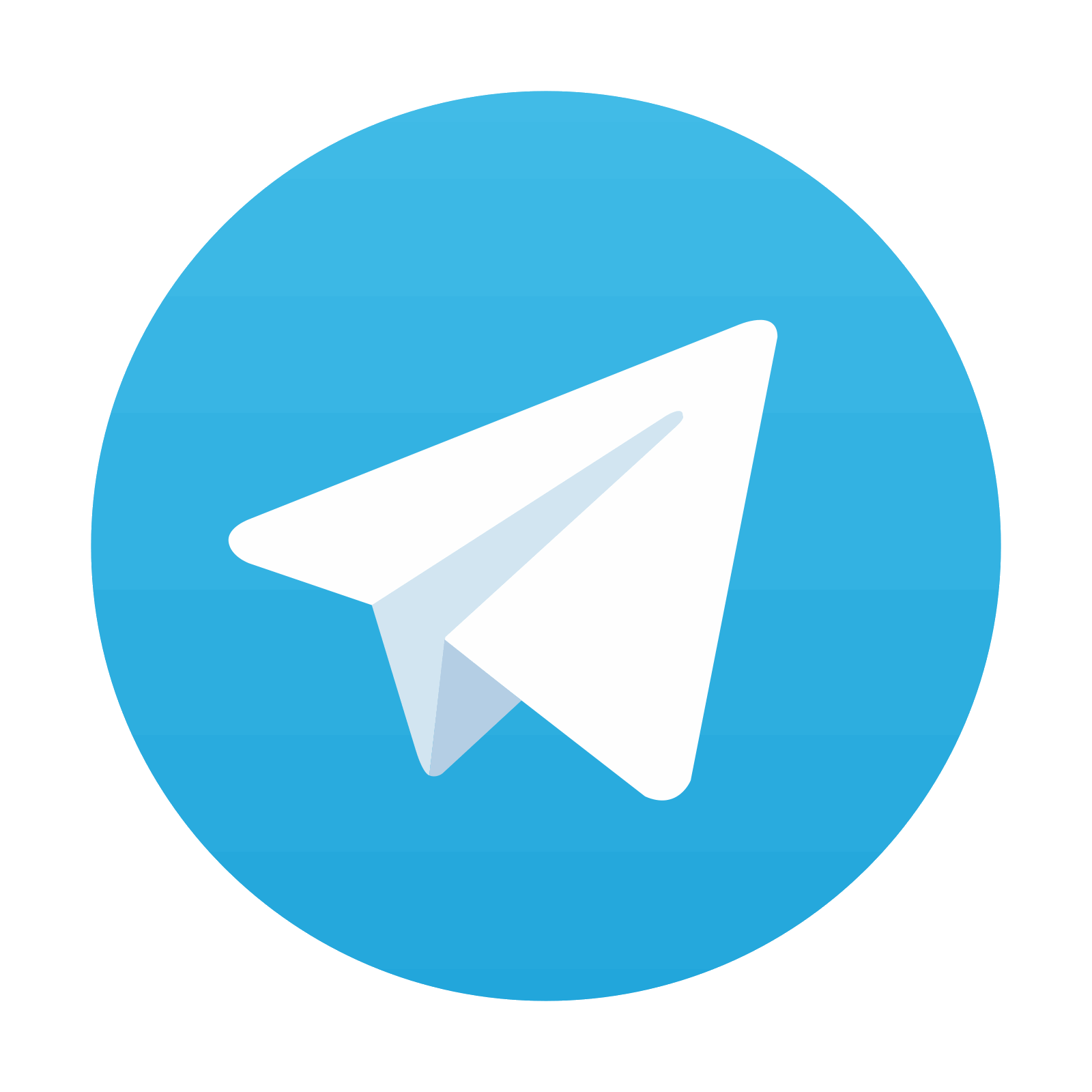
Stay updated, free articles. Join our Telegram channel
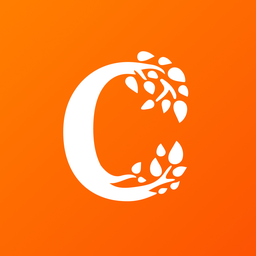
Full access? Get Clinical Tree
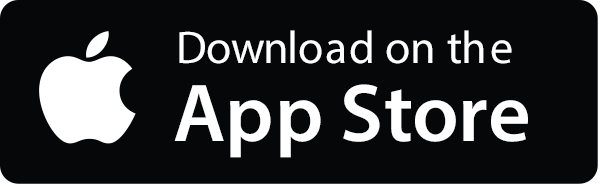
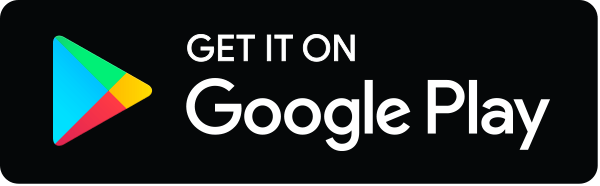
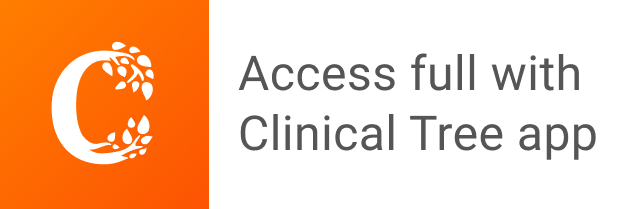