Material
Tensile strength (MPa)
0.2 % Yield strength (MPa)
Elongation (%)
Elastic modulus (GPa)
Reference
316L
595
275
60
193
[1]
Nb
195
105
25
103
[2]
Nb-1Zr
241
138
20
68.9
[3]
Ti
240–331
170–241
30
102.7
[3]
Ti-6Al-4V
900–993
830–924
14
113.8
[3]
The potential for development of further higher-strength alloys is however also well demonstrated in Table 11.1 simply by comparing the superior tensile properties of Nb-1Zr against pure Nb, in this instance the zirconium providing a precipitation strengthening and solid solution strengthening effect. Aside from pure Nb and Nb-1Zr, there are very few commercial niobium materials available; product forms, material specifications and availability of all niobium materials have primarily been influenced by the nuclear and aerospace industries where the corrosion resistance and high temperature performance of the material have been advantageous. A higher-strength Nb-10Hf-1Ti-0.5Zr (commercially known as Alloy C103) was also developed, specifically for the Apollo space program [4], but high costs and commercial restrictions on availability have seen limited access and application development for this material. Despite these challenges, an increasing awareness of the biocompatibility of niobium has seen several positive advances in recent years. This chapter reviews some of the fundamental studies that have increasingly demonstrated the biocompatibility aspects of niobium surfaces. In addition, some of the main developments aimed at improving specific features of mechanical performance are also described.
11.2 Biocompatibility of Niobium
It is widely recognized that the stability of the oxide layer on any metal surface is a good indicator of biocompatibility; in this regard, niobium very readily forms a stable and protective oxide layer. In this context, stability does not simply refer to the absence of corrosion, but also relates to the electrochemical properties of the surface and the nature of current flow between an implant surface and its environment. Early work by Zitter and Plenk [5] studied the relative merits of a number of metallic implant materials, including niobium, by measuring surface current densities under a range of in vitro test conditions. Niobium consistently demonstrated lower current densities than stainless steel, cobalt-chromium and gold, and the authors cite this behaviour as being fundamental to the biocompatibility of the material; the niobium had a performance comparable to pure titanium. This comparability between titanium and niobium has also been demonstrated in vivo by Johansson and Albrektsson [6] for an orthopaedic application. Screw-shaped components of both materials were implanted into the femur and tibia of rabbits. At 3-month follow-up, the removal torque for the niobium components was superior to the titanium components, indicating a good integration of the device within the surrounding bone tissue. While surface roughness was also acknowledged to be influencing this outcome, the histological studies did support the finding with similar levels of bone-to-metal contact observed for both materials. Matsuno et al. [7] have further demonstrated the biocompatibility of niobium in orthopaedic hard tissue environments, as well as in soft subcutaneous tissue. Small wire components, made from niobium, titanium and other refractory metals, were implanted into both the femur and abdominal subcutaneous tissue of Wistar rats. At 4 weeks, newly formed bone was demonstrated on histological sections – the level of osteogenesis on niobium was similar to the titanium. Elemental mapping techniques were also utilized, demonstrating that niobium had not diffused into the surrounding bone tissue. The outcomes of the soft tissue implants were also positive with the components being encapsulated within a thin layer of fibrous connective tissue at 4 weeks, with no inflammatory responses observed.
The electrochemical basis for the performance of niobium has also been demonstrated by Metikoš-Huković et al. [8] with niobium surfaces showing a high resistance to oxide film breakdown in physiological solutions, exhibiting passive behaviour up to 10 V, compared to Ti-6Al-4V which showed film breakdown at 4 V. This is illustrated in Fig. 11.1. Interestingly, this study also showed that the beneficial effects of niobium can be seen even when it is present only as an alloying addition within a material; Ti-6Al-6Nb performed superior to pure titanium and Ti-6Al-4V, and this has been attributed to a reduction in the amount of electronic defects in the oxide due to the presence of niobium. Consistent with these electrochemical findings, Rogers et al. [9] have also shown in vitro advantages of Ti-6Al-7Nb over Ti-6Al-4V. Clinically relevant orthopaedic wear debris was created from both metals, added to human monocyte cell cultures and tested for inflammatory mediators after 48 h. A higher inflammatory response was detected for the Ti-6Al-4V compared to the Ti-6Al-7Nb, and it is proposed that this could contribute to lower bone resorption for devices made from the niobium-containing material. The superior performance of Ti-6Al-7Nb is attributed to the better corrosion resistance of this material.
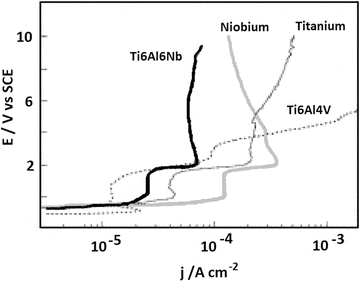
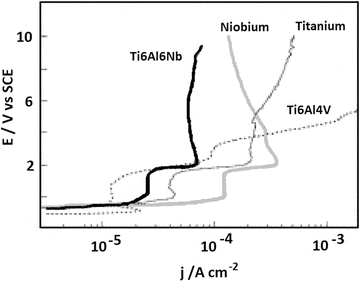
Fig. 11.1
Polarization diagrams for Ti-6Al-4V, Ti-6Al-6Nb, niobium and titanium rotating disc electrodes recorded in HBSS (pH 6.9, T = 37 °C) in the potential range −1.4 and 10 V (Reprinted with permission from Ref. [8]. Copyright 2003 by Elsevier)
In addition to the early in vivo and electrochemical work, the exceptional biocompatibility of pure niobium has also been demonstrated in vitro, by Eisenbarth et al. [10], using cell lines applicable to both orthopaedic and vascular applications. Niobium was compared to a number of metals, including pure titanium, using murine skull osteoblast-like cells and bovine aortic endothelial cells. Proliferation, mitochondrial activity, cell volume and morphology of both cell lines were assessed after 7 days in contact with the metal surfaces. Niobium performed better than titanium in terms of proliferation and mitochondrial activity, while both metals were similar in relation to cell volume and morphology changes.
11.3 Niobium-Based Coatings and Surface Modifications
11.3.1 Sol-Gel Deposition
With the increasing recognition of the excellent biocompatibility of niobium surfaces, and in particular niobium oxides, there have been a number of advances relating to deposition of niobium-based coatings onto substrates with more acceptable mechanical properties, i.e. stainless steel and titanium materials. Eisenbarth et al. [11] have used a sol-gel process to deposit a niobium oxide (Nb2O5) layer onto pure titanium surfaces. The sol was first created using a mixture of niobium(V) ethoxide precursor, a solvent and chelating agent. The substrates were then spin coated and dried, with multiple cycles used to build up the layer thickness to 100 nm. Following drying at 400 °C to remove organic residuals, the samples were given calcination heat treatments in the range 450–700 °C. Different temperatures and times provided oxides with structures ranging from amorphous to crystalline and with surface roughness values (Ra) from 7 to 40 nm, respectively. A range of cell tests were then performed, using murine skull osteoblast-like cells; these tests included cell migration, cell spreading and cell adhesion. Cells showed better migration and adhesion behaviour on the smoother surfaces, with cell spreading being optimum at intermediate roughness levels. This study also explored collagen synthesis by the cells; collagen I synthesis is an essential prerequisite for the bone remodelling process, with the subsequent mineralization of these collagen fibres leading to newly formed bone around an implant. Higher levels of collagen synthesis were observed on the intermediate and roughest surfaces compared to the smooth surface. While this work further demonstrates the potential for niobium surfaces, it also shows that in the case of such a sol-gel process, there is significant scope for tuning of the response.
The use of sol-gel technology to apply niobium oxide (Nb2O5) coatings to titanium has similarly been explored by Ochsenbein et al. [12], in a study that also assessed TiO2, SiO2 and combined TiO2-SiO2. The niobium oxide was created using a niobium(V) ethoxide precursor with acetylacetone as a solvent. The sol was deposited onto the titanium substrate by spin coating; after drying, the deposit was heat treated at 450 °C. The Nb2O5 film produced was amorphous and porous in nature, with a thickness of 130 nm. No detectable process residues were identified in the coating; this will be a key aspect for such sol-gel technologies when considered from a quality assurance and regulatory approval perspective, i.e. being able to demonstrate that such chemical processes do not leave harmful residues. The osseointegration behaviour of these coatings was assessed using disc samples seeded with osteoblasts and incubated for up to 6 days. Proliferation, cell vitality, cell spreading and adhesion were all highly acceptable for the niobium oxide, though it was noted in this instance that higher levels of roughness on niobium oxide can reduce the level of proliferation and spreading. This again demonstrates the importance of being able to tune the biological response through coating design and process controls.
The ability to deposit niobium oxide coatings by sol-gel onto 316L stainless steel has been demonstrated by Nagarajan et al. [13], with the desire to create textured porous surfaces to enhance the formation of a bone-like apatite layer on implants. In this instance, niobium butoxide, ethanol and polyethylene glycol were used as the precursors. Following a dip coating and drying process, the samples were heat treated at 500 °C for 1 h. This study did not include biocompatibility evaluations, with FTIR used to confirm the presence of Nb2O5 and corrosion tests demonstrating that the deposit is providing a complete barrier between the physiological environment and the 316L substrate. This ability to completely seal the substrate from the environment will be another key issue for developing practical devices and applications from these technologies. Substrate ion release could impair the benefit being conferred by the niobium oxide and could also lead to issues with substrate corrosion and coating delamination.
11.3.2 Sputter Deposition and Ion Implantation
Coatings deposited by physical vapour deposition (PVD) techniques would invariably be expected to have higher density and lower porosity than those applied by chemical or electrochemical processes. Therefore, in the context of minimizing pathways between the physiological environment and the substrate metal (with lower biocompatibility than niobium), PVD techniques are also being explored for applying niobium oxide to metals such as stainless steel and titanium. Ramirez et al. [14] describe a magnetron sputtering technique for deposition of 200-nm niobium oxide coatings onto 316L stainless steel substrates. Oxygen was used as the reactive gas constituent in the argon plasma, with oxygen levels kept high enough to ensure Nb2O5 formation, rather than NbO or NbO2; the oxides with lower oxygen contents were conductive and semiconducting and therefore potentially undesirable in terms of characteristics for corrosion resistance and biocompatibility. Coatings were verified to be amorphous, based on x-ray diffraction (XRD) data, while potentiodynamic polarization testing was used to assess the corrosion performance. Oxide-coated samples showed improved corrosion resistance compared to 316L stainless steel, while it was also possible to identify how process conditions, including oxygen content, influenced corrosion resistance. (There is little data to support whether amorphous or crystalline niobium oxides are best from a biocompatibility perspective. In any event, other characteristics such as thickness, topography and porosity are all likely to change as structures shift from amorphous to crystalline, making isolation of crystallinity effects somewhat redundant. Work by Lai et al. [15], on magnetron-sputtered niobium oxides, has shown deposits to be amorphous below 2,010 nm and crystalline above this threshold.)
Further work by Ramirez et al. has taken these niobium oxide surfaces through to a range of in vitro biocompatibility evaluations, with a view to dental applications [16]. In this instance, the objective was to explore lower-cost dental implant materials and designs, i.e. to assess if niobium oxide-coated stainless steel components could compete with titanium. In addition, niobium nitride (NbN) coatings were also prepared – these nitride coatings would be expected to give high hardness and wear resistance [17] and may therefore also be candidates for dental applications. The niobium nitride coatings were also prepared by unbalanced magnetron sputtering, using nitrogen gas as the reactive constituent; deposits of 200 nm were again explored. The biocompatibility evaluations of both coatings were performed using cells derived from a human cementoblastoma. Cementoblasts promote formation of cementum around the teeth roots, which creates anchorage between the teeth and the alveolar bone. Cell adhesion, viability and proliferation were assessed after seeding of cells onto the test surfaces. The niobium nitride showed better adhesion than the niobium oxide, with the Ti-6Al-4V control being better than both. However, cell viability data showed that both coatings are not toxic for human cementoblast cells, while the proliferation data for both was comparable to that of the Ti-6Al-4V, with the niobium nitride slightly better than the niobium oxide. Overall, the investigators propose that both niobium-based coatings are likely to promote cementoblast differentiation and subsequent cementum formation. It is noted that the nitride coatings are crystalline while the oxide coatings are amorphous. Though as indicated earlier, there is not enough evidence to reliably identify if crystalline or amorphous surfaces would ideally be best; these various studies are showing positive outcomes for both, while factors such as inherent porosity, topography and mechanical integrity are likely to be much more significant in the overall performance. This is illustrated in the outcomes of a related study by Rojas and Rodil [18] where amorphous niobium oxide coatings were also created on 316L stainless steel using magnetron sputtering. In this instance, corrosion performance was impaired by coating delamination during immersion in NaCl test solutions. This is attributed to an open porous pathway through the coating, allowing the physiological solution to corrode the substrate, with corrosion product forcing delamination. Such reactions and performance would significantly disrupt overall biocompatibility. Thus even for PVD coatings, physical characteristics such as porosity and topography are likely to be more important in the overall biocompatibility context rather than the degree of crystallinity alone, and just like the sol-gel technology, process understanding and control are key to optimizing coating design.
The use of niobium nitride coatings has also been explored by Park et al. [19] for extending the lifetime of metal-on-polymer artificial joints, by application of the nitride to the metal component. Nitrogen ions were first implanted into the surface of the Co-Cr-Mo alloy to a depth of approx 60 nm using a plasma immersion ion implantation process. This created chromium nitrides (CrN and Cr2N) which contributed to initial surface hardening of the Co-Cr-Mo. The plasma system was then used for deposition of a 600-nm niobium nitride film, using a niobium sputtering target and an argon-nitrogen gas mixture. Wear tests were performed using a pin-on-disc arrangement in distilled water; processed test samples were used as the disc and ultrahigh molecular weight polyethylene (UHMWPE) as the pin. The test data shows that the niobium nitride coating reduces wear of the UHMWPE significantly compared to untreated Co-Cr-Mo. Addition of the nitrogen ion implantation step before application of the coating gives further reductions in wear. Given the in vitro biocompatibility work reported by Ramirez et al. on niobium nitride and the mechanical performance reported here by Park et al., it will be interesting to see if this coating can be progressed next to in vivo preclinical evaluations.
Magnetron sputtering has also been used for deposition of niobium carbide coatings, with exploration of different carbon to niobium ratios [20]. The coatings were applied to Ti-6Al-4V substrates, with methane (CH4) added to the argon as the source of carbon. The mass flow ratio of methane and argon was adjusted to control the carbon content. XPS evaluations showed that the carbon to niobium ratio values were in the range of 0.7–1.9, with nonstoichiometric NbC phases present. The coatings were primarily crystalline in nature, though at the highest C:Nb ratio (1.9), these crystalline structures coexisted with an amorphous carbon phase. Corrosion testing showed that while all coatings exhibited improved corrosion behaviour compared to Ti-6Al-4V, the composite structure with the amorphous carbon phase performed best; this structure also had the lowest porosity, again pointing to the importance of such physical characteristics. Biocompatibility was evaluated using osteoblasts (derived from a human osteosarcoma) for cell viability tests, after contact with the test surfaces for 5 days. There was no significant difference between the various niobium carbides and the Ti-6Al-4V, but the coating with the highest C:Nb ratio was showing the highest cell viability. While it is difficult to compare these carbide coatings directly with the oxides or nitrides, these initial results nevertheless show significant merit.
Coatings of pure niobium (not oxides, nitrides or carbides) have also been applied to 316L stainless steel using the same unbalanced magnetron sputtering techniques, and these have been evaluated for both corrosion performance and biocompatibility [21]. In this instance, cell culture evaluations have been performed using osteoblasts derived from human alveolar bone. Cell adhesion, viability, proliferation and morphology were assessed after seeding onto the niobium-coated samples and 316L stainless steel controls. The niobium-coated surfaces performed superior to the stainless steel in all evaluations. The morphology evaluation is more qualitative in nature but shows that the actin cytoskeleton organization occurs faster and more efficiently on the niobium compared to the stainless steel. The niobium promotes extension of the cell projections compared to the stainless steel where the osteoblasts retained a more rounded appearance. While pure niobium surfaces are not likely to be as hard as oxides, nitrides or carbides, these pure metal surfaces still probably have some degree of niobium oxide present and in this condition will confer sufficient biocompatibility and hardness for some applications.
Implantation of niobium into the surface of nitinol (NiTi) has been explored by Zhao et al. [22] primarily to assess the impact it may have on corrosion performance of the NiTi. Samples of NiTi were first argon ion sputtered to clean the surface, and then the Nb ion implantation was performed using a metal vapour vacuum arc plasma source. Based on Auger electron spectroscopy (AES) and X-ray photoelectron spectroscopy (XPS) analysis, it is interpreted that a composite Nb2O5/TiO2 film of approximately 30-nm thickness forms during the implantation process; nickel surface concentration is reduced. It is noted that the implantation process roughens the surface. Corrosion data, as determined by potentiodynamic polarization, show that the Nb-implanted NiTi has a higher breakdown potential (>1,000 mV) compared to the untreated NiTi (420 mV). In addition the Nb-NiTi exhibits lower corrosion current density as shown in Fig. 11.2. Overall, it would be expected that these improvements in corrosion performance should also lead to improved biocompatibility. In terms of the functional performance of the NiTi, further evaluations would also be needed to verify if the shape memory or superelastic response has been affected – at a scale relevant to practical medical devices. This is particularly important as this study showed that some softening and modulus reduction was observed beyond the depth of the implanted niobium ions.
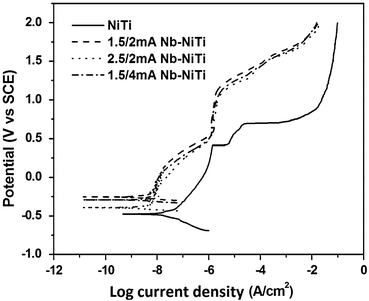
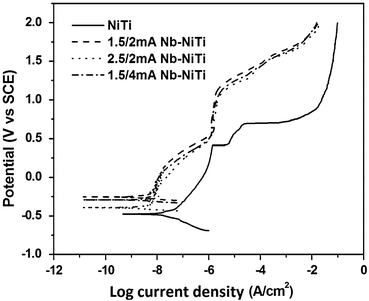
Fig. 11.2
Potentiodynamic anodic polarization curves for untreated NiTi and ion-implanted samples (Reprinted with permission from Ref. [22]. Copyright 2011 by Elsevier)
11.3.3 Alkali Treatment for Apatite Formation
The low elastic modulus of niobium makes it particularly attractive in terms of the potential for reducing stress shielding effects in large load-bearing implants. While the compatibility of pure niobium with bone formation and integration processes is already described, efforts to enhance this have also been explored through surface modifications that enhance the creation of bone-like apatite layers in the physiological environment. Goldley et al. [23] report on the formation of apatite structures on pure niobium in simulated body fluid (SBF), after pretreatment in alkaline solutions. Samples were soaked in NaOH solutions of different concentrations and at different temperatures for 24 h. A treatment using 0.5 M NaOH at 25 °C for 24 h produced well-adhered porous surface structures, with an average pore size of around 100 nm. Based on AES and EDS data, the investigators interpret this porous layer to be a sodium niobate hydrogel. After soaking the NaOH-treated samples in SBF for 6 days, a layer of spherical grains is observed to form. Calcium, phosphorus and oxygen are detected in this structure, indicating the layer to be calcium phosphate – the morphology is similar to calcium phosphate layers formed on titanium and titanium alloys. Below the calcium phosphate, an intermediate layer containing calcium, niobium and oxygen is observed, and this is deemed to be calcium niobate – comparable calcium titanate layers are reported for titanium surfaces. The calcium phosphate layer is identified to be amorphous, and in this regard it is similar to the initial calcium phosphate deposits that precipitate during the formation and maturing of natural bone apatite. It is therefore possible that such NaOH treatments could further enhance the suitability of niobium for orthopaedic implant applications.
Wang et al. [24] have explored a variation of the alkali treatment step. Pure niobium samples were immersed in 0.5 M NaOH and heated to 60 and 80 °C, in a sealed container, for 24 h. After washing, the samples were vacuum heat treated for 1 h at 600 °C. The treated surface exhibited a porous fibre-like structure with smaller diameters (50–100 nm) obtained at 80 °C than at 60 °C. This structure was identified to be crystalline sodium niobate (NaNbO3). After immersion in SBF for 3 weeks at 37 °C, a dense globular-like film had deposited on the surface; the 80 °C treatment provided better coverage than the 60 °C treatment. Control surfaces, without alkali treatment, exhibited no apatite formation. While not described specifically in this study, it is likely that the 600 °C treatment should enhance adhesion of the apatite layer to the substrate. Similar treatments and approaches are already developed for titanium alloys [25], though much more work will be needed for niobium to understand adhesion and durability aspects in the context of in vivo device deformations and deformations/damage during implantation.
11.3.4 Anodic Oxidation of Niobium
Anodic oxidation has been explored for creating porous structures on a range of metals with the objective of increasing surface area, in applications such as sensors, catalysts and capacitors. In relation to medical devices, the approach is of interest to create surfaces and reservoirs for attachment of drugs or biological structures, to promote tissue integration [26] and to potentially improve surface hardness (scratch resistance) and corrosion performance. As niobium also has application across a number of sectors, it is noted that much of the applicable research into anodic oxidation is coming from other industries and not just from the biomedical field; fundamentally, this research is still of high significance to medical device applications. Sieber et al. [27] describe a process for anodic oxidation of pure niobium in sulphuric acid (H2SO4), resulting in the formation of a highly ordered porous niobium oxide structure. The 1-M H2SO4 solution contained small quantities (0.5–2.0 wt%) of hydrofluoric acid (HF), and all anodization treatments were performed at room temperature. Samples were anodized at 20 V (relative to the mercury sulphate electrode) for different durations. Porous structures were obtained for a wide range of process conditions with, for example, pores of approximately 20–30 nm obtained at 20 V for 30 min with 0.5 % wt HF as illustrated in Fig. 11.3. Thickness typically increases with anodizing duration and also depends on HF concentration; values in the range of 100–500 nm were recorded. The films were confirmed to be Nb2O5.
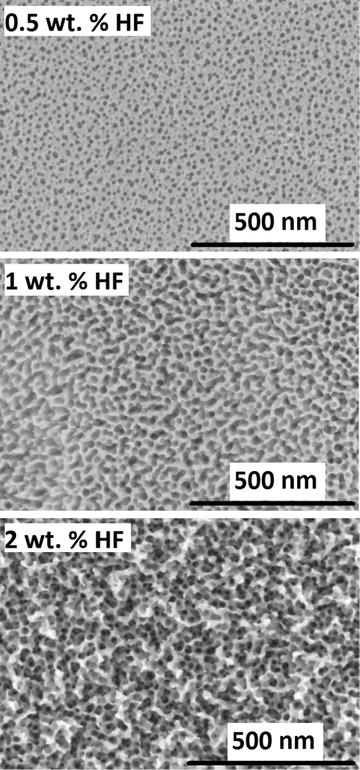
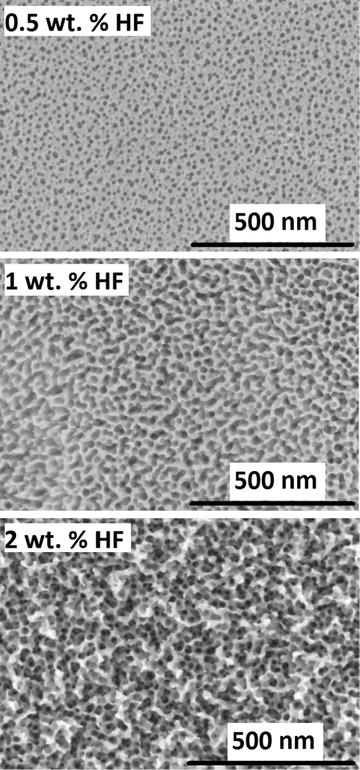
Fig. 11.3
SEM images of porous niobium oxide layers formed in 1 M H2SO4 with different concentrations of HF after 30-min polarization at 20 V (Reprinted with permission from Ref. [27]. Copyright 2004 by Elsevier)
Karlinsey [28] has also explored anodization of niobium, but in this instance using HF solutions (0.25–2.5 wt%), without H2SO4. A wide range of voltages, temperatures and durations were examined, with unusual conical-shaped niobium oxide structures formed under various conditions. The structures are relatively large with cone base diameters in the range 5–20 μm; though the growth process is such that sharp, well-defined tips with nanometre resolution are obtained. Higher voltages lead to a higher density of the conical structures on the surface. While no mechanical or in vitro testing is reported, such structures could also be interesting for modifying cell responses or for attaching of drugs or biologics – similar to the tubular porous structures already described.
An interesting anodization process from the electrical capacitors industry, which creates another unusual morphology, is described by Störmer et al. [29]. Using powder metal technologies, pure niobium powder is pressed and then vacuum sintered giving a porous, sponge-like structure. Samples were then anodized in a two-stage process, an initial galvanostatic step with constant current flow, followed by a potentiostatic step at 40 V. The process was carried out in a 1 mass% aqueous solution of phosphoric acid at 65 °C. After anodizing, samples were annealed at temperatures up to 320 °C. While this study naturally focuses on the electrical characteristics (and the annealing step in particular is a key aspect in that regard), the microstructure obtained could have biomedical applications; the sponge-like structure of niobium is surrounded by an amorphous layer of niobium oxide (Nb2O5). Thus from a cell and tissue integration perspective, it is potentially a biocompatible porous construct but with integrity and strength provided by the niobium substrate.
In a manner comparable with the sol-gel and sputtering techniques already described, the use of anodization to create niobium oxides on metallic substrates, other than niobium, has also been explored. This can be achieved by first applying a thin layer of niobium to the substrate as described by Mackey et al. [30] in the case of titanium dental implant screws. In this instance, a 5-μm coating of niobium was sputter deposited onto sand-blasted titanium screws. These components were then anodized using the HF solution methods of Karlinsey (reviewed earlier). Careful process optimization was needed, balancing between promotion of niobium oxide growth and delamination of the niobium interlayer from the substrate; an anodizing duration of 1 h, with a 0.1 wt% HF solution, provided an optimum balance, resulting in a surface considered suitable for in vivo evaluations in the next phase of the work.
11.3.5 Boronizing of Niobium
Boronizing has been widely used for improving the hardness and wear resistance of many metallic materials; diffusion of boron atoms into the surface allows for the formation of hard boride phases. Usta [31] has investigated the process for pure niobium, with the aim of increasing surface hardness. The process was performed by surrounding the test samples in commercial boronizing powder (90 % SiC, 5 % B4C and 5 % KBF4) and soaking at 940 °C for durations of up to 8 h. The thickness of the boride layer formed was directly proportional to the soak duration, with the 8-h treatment resulting in a 22-μm boride layer. This layer consisted primarily of NbB2 with a Vickers hardness (50 g) of 2,500 HV – this compares to a hardness of 110 HV for the niobium substrate itself. An intermediate layer also existed between the substrate and boride layer; this had a hardness of 670 HV with this increase in hardness being attributed to solid solution strengthening by the boron. These coatings have been evaluated for friction and wear performance in a subsequent study by Ribeiro et al. [32]. The tribological tests were carried out with a reciprocal pin-on-disc tribometer under dry conditions and also with simulated body fluid (SBF). The data shows a favourable outcome in SBF, with a coefficient of friction at 0.2 compared to 0.6 for the dry conditions. Less wear debris was also generated when tested with SBF. While this is a promising surface technology, with potential for orthopaedic wear-resistant applications, more work will be needed to evaluate this performance in comparison to requirements for specific targeted applications and to establish biocompatibility responses. A separate study by Dokumaci et al. [33] does however demonstrate that niobium boride surfaces have good oxidation and degradation resistance – this is at least a good starting position with regard to potential in vivo behaviour.
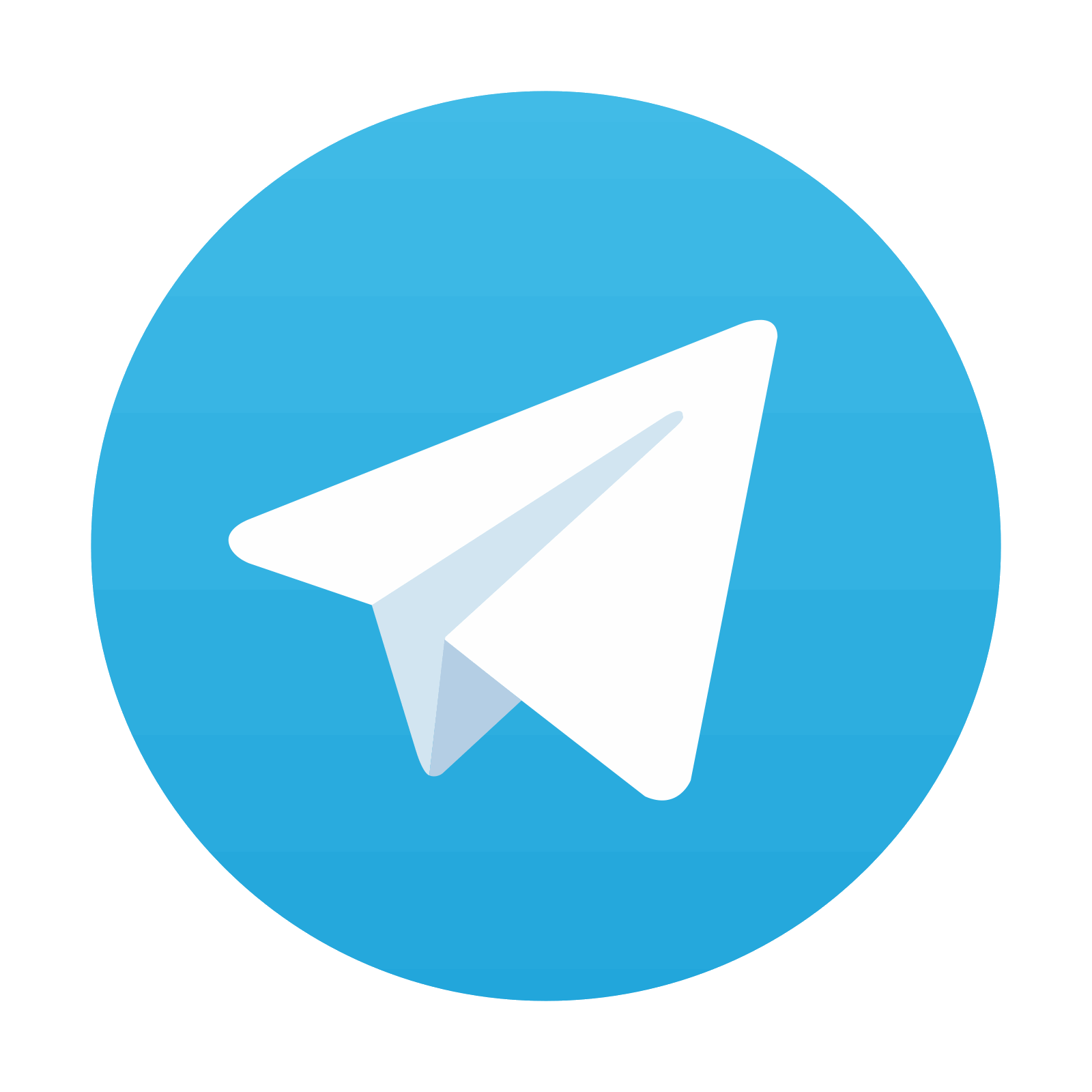
Stay updated, free articles. Join our Telegram channel
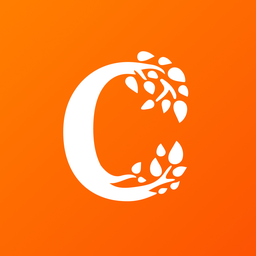
Full access? Get Clinical Tree
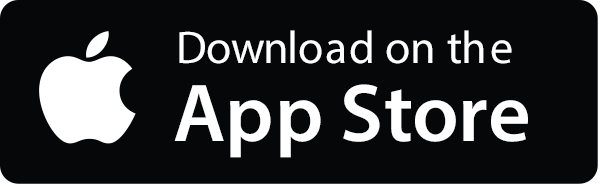
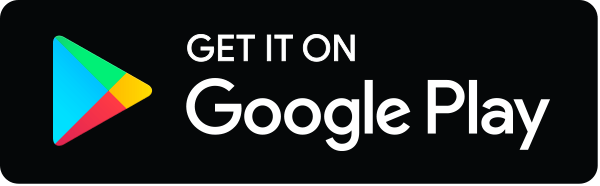