Standard
Specifications
ASTM F746
Standard Test Method for Pitting or Crevice Corrosion of Metallic Surgical Implant Materials
ASTM F897
Standard Test Method for Measuring Fretting Corrosion of Osteosynthesis Plates and Screws
ASTM F1089
Standard Test Method for Corrosion of Surgical Instruments
ASTM F1801
Standard Practice for Corrosion Fatigue Testing of Metallic Implant Materials
ASTM F2129
Standard Test Method for Conducting Cyclic Potentiodynamic Polarization Measurements to Determine the Corrosion Susceptibility of Small Implant Devices
ASTM G5
Standard Reference Test Method for Making Potentiodynamic Anodic Polarization Measurements
ASTM G31
Standard Guide for Laboratory Immersion Corrosion Testing of Metals
ASTM G48
Standard Test Methods for Pitting and Crevice Corrosion Resistance of Stainless Steels and Related Alloys by Use of Ferric Chloride Solution
ASTM G61
Standard Test Method for Conducting Cyclic Potentiodynamic Polarization Measurements for Localized Corrosion Susceptibility of Iron-, Nickel-, or Cobalt-Based Alloys
ASTM G71
Standard Guide for Conducting and Evaluating Galvanic Corrosion Tests in Electrolytes
In vitro corrosion studies on orthopedic biomaterials are carried out either in Hanks’ solution or Ringer’s solution whose constituents are given in Table 12.2, comparatively, whereas the corrosion resistance for dental materials is evaluated using synthetic saliva whose constituents are shown in Table 12.3 [52–54]. Furthermore, corrosion changes the chemical environment around the implant, inducing an acidic pH after the inflammation of the implant and thus increasing the likelihood of corrosion.
Substance | Hank composition (g ∙ L−1) | Ringer composition (g ∙ L−1) |
---|---|---|
NaCl | 8.00 | 8.69 |
KCl | 0.40 | 0.30 |
CaCl2 | – | 0.48 |
NaHCO3 | 0.35 | – |
NaH2PO4 ∙ H2O | 0.25 | – |
Na2HPO4 ∙ 2H2O | 0.06 | – |
CaCl2 ∙ 2H2O | 0.19 | – |
MgCl2 | 0.19 | – |
MgSO4 ∙ 7H2O | 0.06 | – |
Glucose | 1.00 | – |
pH (~) | 6.90 | 6.4 |
Components | Xialine 1 (g ∙ L−1) | Xialine 2 (g ∙ L−1) | Saliveze (g ∙ L−1) |
---|---|---|---|
Xanthan gum | 0.92 | 0.18 | – |
Sodium carboxymethylcellulose | – | – | 10 |
Potassium chloride | 1.2 | 1.2 | 0.62 |
Sodium chloride | 0.85 | 0.85 | 0.87 |
Magnesium chloride | 0.05 | 0.05 | 0.06 |
Calcium chloride | 0.13 | 0.13 | 0.17 |
Dipotassium hydrogen orthophosphate | 0.13 | 0.13 | 0.80 |
Potassium dihydrogen orthophosphate | – | – | 0.30 |
Sodium fluoride | – | – | 0.0044 |
Sorbitol | – | – | 29.95 |
Methyl p-hydroxybenzoate | 0.35 | 0.35 | 1.00 |
Spirit of lemon | – | – | 5 ml |
The pH of normal blood and interstitial fluid is 7.35–7.45. Acidification increases metal ion release, especially below pH of 4. In this state, the Japanese Industrial Standards (JIS) [55] can be used for comparison of metallic biomaterials in the acidic simulating in vivo environment.
12.5 Corrosion of Stainless Steels
Stainless steels used in biomedical applications differ in microstructure and chemical composition, and they are classified mainly as martensitic, ferritic, and austenitic stainless steel with respect to their microstructures [7, 9, 14, 56]. On the other hand, according to chemical composition, martensitic and ferritic stainless steels are grouped as chromium and carbon alloys, while austenitic steels fall into chromium–nickel-type stainless steel group.
In medicine the austenitic stainless steels (AISI 316 L, ASTM F55, and F138) contain Cr (17–20 mass%), Ni (12–15 mass%), Mo (2–3 mass%), and small amounts of other elements. The letter “L” represents low carbon (<0.03 %) which is effective in lessening the intergranular corrosion. Cr is responsible for high passivation and it increases the resistance against localized breakdown of passivity. Likewise, Mo reduces the size and number of metastable pits [57]. Accordingly, 316 L stainless steels are desirable surgical implant materials as they additionally possess acceptable biocompatibility and suitable density for load-bearing applications [56]. Commonly used stainless steels in medical and surgical applications are presented in Table 12.4.
Table 12.4
Standards related to surgical stainless steel implants
Specification | Nominal contents |
---|---|
F 138-97 | Wrought 18Cr–14Ni–2.5Mo, bar, and wire |
F 139-96 | Wrought 18Cr–14Ni–2.5Mo, sheet, and strip |
F 745-95 | 18Cr–12.5Ni–2.5 Mo, cast, and solution annealed |
F 899-95 | Stainless steel billet, bar, and wire |
F 1314-95 | Wrought N strengthened, 22Cr–12.5Ni–5Mg–2.5Mo, bar, and wire |
F 1586-95 | Wrought N strengthened, 21Cr–10Ni–3Mg–2.5Mo, bar |
Although the carbon content of traditional austenitic stainless steels like 316 and 317 L is below 0.03 % and Mo addition is around 2–3 %, they still do not fully meet the desired corrosion resistance in the case of a localized form of corrosion. In addition, austenitic stainless steels containing nickel cause a negative reaction when implanted into the body and may induce harmful effects due to the release of nickel ions [58–60], which can be toxic to the human body. Because of these, in recent years, nickel-free nitrogen-containing FeCrMnMoN stainless steels, Table 12.5, with non-ferromagnetic properties are considered as potential replacement for Ni-containing austenitic stainless steels [60–64].
Table 12.5
Nitrogen-containing nickel-free austenitic stainless steels (mass %)
Year | Chemical composition |
---|---|
1996 | Fe-18Cr-18Mn-2Mo-0.9N |
1996 | Fe-15Cr-(10–15)Mn-4Mo-0.9N |
1996 | Fe-(15–18)Cr-(10–12)Mn-(3–6)Mo-0.9N |
2000 | Fe-17Cr-10Mn-3Mo-0.5N-0.2C |
2001 | Fe-(19–23)Cr-(21–24)Mn-(0.5–1.5)Mo-(0.85–1.1)N |
Nitrogen has been reported to increase austenite and passive film stability and also the resistance to the localized form of corrosion [34, 35, 65]. In nickel-free austenitic stainless steels, as a nickel-substituting element, manganese is also used in addition to nitrogen [33]. Accordingly, nickel-free stainless steels with lower toxicity can solve the “nickel problem” in medical stainless steels and are developed as the next generation of metallic implants [14, 56].
High nitrogen content may cause precipitation of nitrides and degrade the corrosion resistance due to localized chromium depletion. Moreover, increased nitrogen content increases brittleness of the steel and ductile to brittle transition [56]. To avoid brittleness, usually nitrogen content is tried to be kept below 0.9 mass% for medical applications.
The passivity of stainless steels is enhanced by modifying the chemical composition and morphology of the oxide layer [66]. The oxide film formed on surgical steels is generally rich in Cr2O3 [67, 68]. In fact, the metal ion release from different grades of stainless steels depends on the Cr content of the film, which is responsible for passive film formation. It has been shown that with the increase in Cr/(Cr + Fe) ratio in passive film, the metal ion release from stainless steel into artificial lysosomal fluid decreases [69–71].
In general, the passivation process applied to stainless steels like thermal, electrochemical, and nitric acid treatment changes the composition of the oxide. Depending on the process, Cr-rich oxide [72, 73], Fe-rich oxide [74], and mixture of Fe and Cr oxide [75], Fe-Cr oxyhydroxide [76], or hydrated Cr-rich oxyhydroxide are obtained.
In addition to types of surface oxides, investigations have shown that thickness of the oxide layer plays a lesser role than the uniformity and structure of the oxide layer. Studies carried out on 316 L stainless steel have revealed that amorphous oxide film has higher corrosion resistance compared to the polycrystalline oxide layer [77]. The surface film crystalline defects act as active sites for corrosion. The absence of grain boundary, defects, and dislocations on amorphous oxide film provide the lowest current density and absence of breakdown potential, which minimizes the release of metallic ions [79, 80].
Chromium, molybdenum, nitrogen, and titanium in stainless steels are adjusted to improve corrosion resistance. For example, in a chloride-containing solution, the corrosion resistance of stainless steels is improved by the presence of Cr and Mo in the steel. Mo inhibits corrosion process by increasing the stability of passive film due to formation of protective molybdenum oxide film beneath the hydrated chromium-rich oxide film [2, 14]. The addition of titanium and nitrogen elements also improves the corrosion resistance of stainless steels. Corrosion studies conducted in Hanks’ solution [15, 81] exhibited higher resistance for titanium-modified 316 L stainless steel compared to the commonly used 316 L, which was attributed to the enrichment of chromium and bound water in the form of OH− ions at the outermost layer of the passive film. Likewise, addition of nitrogen increases resistance to pitting corrosion of stainless steels by dissolution of nitrogen at the pit site and subsequent formation of ammonium ions NH4+ and NO3− in the metal/film interface which increases pH and thus slows down pit growth kinetics [82]. As well as the nature of the film, the presence of inclusions like MnS in stainless steel induces pitting corrosion since pits initiate on the inclusions.
12.6 Corrosion of Titanium and Its Alloys
Titanium and its alloys are frequently used in biomedical applications due to especially their good corrosion resistance, biocompatibility, greater specific strength, and much lower elastic modulus than the other metallic biomaterials. The applications of titanium and its alloys cover dental implants and parts for orthodontic surgery, joint replacement parts, bone fixation materials, housing device for the pacemakers and artificial heart valves, surgical instruments, and components in high-speed blood centrifuges [4, 83, 84]. Unlike many other types of materials, there is no general rule for corrosion resistance of titanium and its alloys such that they may corrode either very quickly or extremely slowly depending on the material properties and medium characteristics.
Table 12.6 summarizes the commonly used biomedical titanium and its alloys. Apart from the listed titanium alloys, the equiatomic intermetallic compound of TiNi which exhibits shape memory and superelastic properties is also used as biomaterial for fixation of bone fragments, anchoring of implants and dentures to the living tissues, and poisoning of tissues [85].
Table 12.6
Commonly used biomedical titanium alloys
Material | Standard | Alloy type |
---|---|---|
Commercially pure Ti (CP grades 1–4) | ASTM 1341 | α |
Ti-6Al-4V ELI (wrought) | ASTM F136 | α + β |
Ti-6Al-4V ELI (standard grade) | ASTM F1472 | α + β |
Ti-6Al-7Nb (wrought) | ASTM F1295 | α + β |
Ti-5Al-2.5Fe | – | α + β |
Ti-13Nb-13Zr (wrought) | ASTM F1713 | Metastable β |
Ti-12Mo-6Zr-2Fe (TMZF) | ASTM F1813 | β |
Ti-35Nb-7Zr-5Ta (TNTZ) | – | β |
Ti-29Nb-13Ta-4.6Zr | – | β |
Ti-35Nb-5Ta-7Zr-0.4O (TNZTO) | – | β |
Ti-15Mo-5Zr-3Al | – | β |
Ti-Mo | ASTM F2066 | β |
Titanium is known for its high corrosion resistance due to instant formation of an inert oxide layer on its surface. Titanium commonly consists of mainly TiO2 in Ti alloys and corrosion resistance of the titanium results from this native titanium oxide [12, 67, 68, 86–90]. The film on titanium, which is typically 3–7 nm thick, consists of amorphous or crystalline anatase or rutile (TiO2) with tetragonal structure. O to Ti concentration ratio varies gradually from 2 to 1, from the TiO2 film to a much lower ratio in the bulk [85, 91–97].
The most stable form of titanium is the oxidation state IV (Ti4+). However, when it reacts with water, hydrated titanium dioxide (TiO2 ∙ nH2O) is seen as the stable product other than Ti(OH)4. Since these processes are pH and potential dependent, the pH and potential values of physiological systems should be considered to determine whether the passivation is achieved or not in vitro or in vivo. Pourbaix diagrams are useful in determining the passivity formation under various combinations of pH and potentials.
Although it is reported that the passive layers on Ti alloys are not different from that on pure Ti [98], some authors [99–102] state that the oxide layer in α- + β-alloys, like Ti6Al4V or Ti6Al7Nb, contains oxides of the alloying elements. For example, relatively higher corrosion resistance of Ti6Al7Nb compared to Ti6Al4V alloy is attributed to the formation of Nb2O5, which is chemically more stable, less soluble, and more biocompatible compared to V2O5 formed on Ti6Al4V alloy [4]. The rate of ion transfer, stability of the oxide film against dissolution, and spontaneous regeneration in milliseconds even after damage determine the protectiveness of the passive film. The presence of defects, thickness, structure, and chemical composition of the film influence the ion transport. On the other hand, the nature and stability depend on medium conditions such as temperature and time of exposure, pH, and composition of the fluid surrounding the metal and redox reactions [7]. For example, TiO2 is thermodynamically stable in the pH range between 2 and 12, and only complexing species, such as HF or H2O2, lead to substantial dissolution. Thus, the oxide film becomes thermodynamically unstable and dissolves if the interface potential is made negative or pH is made low, and this results in the dissolution of the oxide layer. Additionally, the corrosion resistance of the passive film is altered by the variation in the ionic or electrical conductivity of the film or by the structural changes in the film due to alloying elements. For example, by dissolution of vanadium oxide in Ti6Al4V alloy, vacancy generation and diffusion occur in the oxide layer [103]. However, Ti-Ta alloys exhibit relatively better corrosion resistance compared to Ti6Al4V by reducing the concentration of metal release as a result of strengthening the TiO2 passive film with the aid of a more stable Ta2O5 passive film. Similarly, the surface film of Ti-based alloys is stabilized when Nb alloying element is used. The formation of highly stable Nb-rich oxide enhances corrosion resistance, and by the Nb addition, an improvement in the passivation property of the surface film is detected by decreasing the concentration of anion vacancies [104].
Titanium and its alloys show very high pitting potentials in chloride-containing solutions. Therefore, stable pitting corrosion is not a relevant failure mode for these materials in biomedical applications since the relevant potential region in the body is clearly <1 V. Nevertheless, metastable pitting corrosion has been observed for CP Ti and for the Ti6Al4V implant alloy in simulated physiological solutions [105]. Under certain conditions due to wear and fretting and/or due to biochemical factors, localized breakdown of passivity takes place. As a result of corrosion, ion release occurs if the destroyed oxide film remains unrepaired. The amount of metallic ions released during the period depends on the regeneration rate of the film. Spontaneous regeneration of the oxide layer in milliseconds even after damage makes titanium advantageous over the other metallic materials [106–108]. However, ions are detected in the tissue around a titanium implant [109–113] mainly due to continuous activation/repassivation which resulted from combined mechanical and chemical effects. Additionally, titanium ions may also be released from the separately implanted bone plate and screws due to biochemical factors like amino acids and proteins [111–115].
Under in vivo conditions, transient microscopic breakdown of the oxide layer was described to occur in Ti alloys [116, 117]. CP Ti exhibits lower current densities than TiAl6V4 and several β- and near-β-alloys [116]. Only the Ti-30Ta alloy (Ti30Ta) shows a value near that of CP Ti. Either way, there are reactions between the surface and the surrounding liquid, which lead to a thickening of the oxide layer in vivo [94, 118]. These reactions result in a release of species containing Ti and/or, possibly, alloying elements, into the surrounding tissue and in the adsorption of oxygen-containing species from the biological liquids to the surface. The corrosion resistance of CP Ti, the α + β-alloy, TiAl6V4, and various β- and near-β-alloys could be improved by increasing the oxide thickness to around 100 nm, independent of the oxidation procedure [117, 119]. The release of alloying elements can lead to toxic, allergic, and idiosyncratic symptoms. For the Ti-based (TiAlV, TiAlNb, Ti15Zr4Nb4Ta) alloys, mostly Ti is released, but for TiAlV alloys, significant amounts of Al and a small amount of V could be detected. Since vanadium is toxic in the elemental state, new beta titanium alloys with nontoxic alloying elements like Ta, Nb, and Zr have been developed. Although the amounts of Ti and Al release are similar for both TiAlV and TiAlNb alloys, the quantity of Ti released from the TiZrNbTa alloy is much smaller than the quantity released from the TiAl–(V, Nb)-type alloys [7]. It is reported that the addition of Ta remarkably reduces the concentration of the metal release [44, 120–122]. Survival of released ions for a long time in the body fluid and the type of species combined to release metallic ions are important from a toxic point of view. The biomolecules do not contain titanium and comparatively large protein and enzyme molecules hardly react with titanium ions. Titanium oxide and salts are immediately formed as a result of reacting the titanium ions with water molecules and anion species. However, formation of complexes with organic ions such as amino acids survives in body fluids and has a high possibility of combining with biomolecules [123].
Nickel, at certain concentrations, leads to severe local tissue irritation, necrosis, and toxic reactions. However, in vivo and in vitro studies of NiTi implants show excellent biocompatibility [124–126]. There are few reports on release of Ni, but the amount of released nickel is not sufficient to induce such reactions [127]. Titanium alloys tend to repassivate faster than the stainless steel and other biomedical alloys; however, repassivation of titanium in vivo is slower than in vitro. The type of ions in the repassivated layer plays a deciding factor for corrosion resistance. The composition of the surface film changes with environment in which it is existing [128]. For example, after mechanical destruction, the regenerated surface oxide film on titanium in Hanks’ solution was observed to contain calcium phosphate in the outer layer [128].
Apart from the regeneration after mechanical damage, the surface oxide of titanium is not always stable, and its composition changes through the incorporation of ions and molecules in vivo. Similar to passive film on stainless steel, titanium oxide film near the body fluid dissolves partially and reprecipitates. Calcium, phosphorus, and sulfur are incorporated in the film on titanium. As a result calcium phosphates are precipitated on titanium and its alloys in a simulated body fluid [95, 129–131]. Proteins are also incorporated into the reconstructed surface oxide in addition to calcium, phosphorus, and sulfur [132]. There are several methods like thermal, anodic oxidation and the sol–gel process which aim in thickening the oxide layer to get around 100 nm thickness, thereby optimizing the resistance against mechanical damage and corrosion [119, 133–136]. The methods change the chemical composition along with the structure of the oxide layer. Although thermal oxidation induces predominantly TiO2 in the rutile structure for pure titanium and titanium alloys, in certain β-alloys, mixed oxides of titanium are observed. On the other hand, in electrochemical methods depending on the anode potential and type of electrolyte solution, either amorphous or crystalline oxides are formed [119, 136]. Though titanium and its alloys are highly resistant to pitting corrosion under different in vivo conditions, they undergo corrosion in high-fluoride solutions in dental cleaning procedures [137].
12.7 Corrosion of Cobalt-Based Alloys
By the early 1930s, a cobalt–chromium (CoCr) alloy, called Vitallium, was introduced to dentistry as an alternative to gold alloys. CoCr alloys have been used as dental and orthopedic implant materials for fabrication of hip prostheses and internal fixation plates. The wear resistance of CoCr alloys is better than that of stainless steel and titanium (Ti) alloys and it has been shown that their corrosion resistance is better than that of stainless steel. The main disadvantage of CoCr alloys is their low ductility. The chromium enhances corrosion resistance as well as solid solution strengthening of the alloy. In orthopedics, CoCr–molybdenum (Mo) alloys are used for the head and stem of artificial joints, and CoCr–tungsten (W)–nickel (Ni) alloys (HS25) function as bone fixation wires. In dentistry, CoCrMo alloys, CoCrNi alloys, and CoCrNi–copper (Cu) alloys are applied for denture bases, and CoCr–iron (Fe)–Ni alloys are utilized for orthodontic wire.
The CoNiCrMo alloys are relatively new, now used for making the stems of prostheses for heavily loaded joints. The molybdenum is added to produce finer grains, which results in higher strengths after casting or forging. 5–7 wt% Mo in the alloy provides solid solution strengthening and good localized corrosion resistance. The alloy has an oxide layer on its surface, which forms a thin passive film that is enriched with chromium that gives very good corrosion resistance [138–141]. The CoNiCrMo alloy, originally called MP35N (Standard Pressed Steel Co.), contains approximately 35 mass% Co and Ni each. The alloy is highly corrosion resistant to seawater (containing chloride ions) under stress. The superior fatigue and ultimate tensile strength of the CoNiCrMo alloys make it suitable for the applications, which require long service life without fracture or stress fatigue. In the cardiovascular field, CoCr alloys are used for guide wires, while CoCrFeNi alloys, CoNiCrMo alloys (MP35N), and CoCrNiWFe alloys are used for stents. CoCr–tantalum (Ta)–Ni alloys and CoCrNiMoFe alloys are used for aneurysm clips [142]. Consequently, the alloys have become one of the three major biomedical metallic materials and the alloys have been used for many decades in dentistry and, relatively recently, in making artificial joints [143, 144].
Lin et al. [145] investigated the changes in surface oxides of the CoCrMo alloy by biological and cellular environments. Results show that increase in alloy surface oxides increases in vivo corrosion resistance of the alloy. Chromium oxides increase the corrosion resistance of CoCrMo alloys in which heat and nitric acid passivation treatments enhanced alloy surface oxide thickness and raised the O and Cr contents to reduce corrosion [146, 147]. The biologically mediated modifications in surface oxides may affect corrosion of the implant devices [148–150].
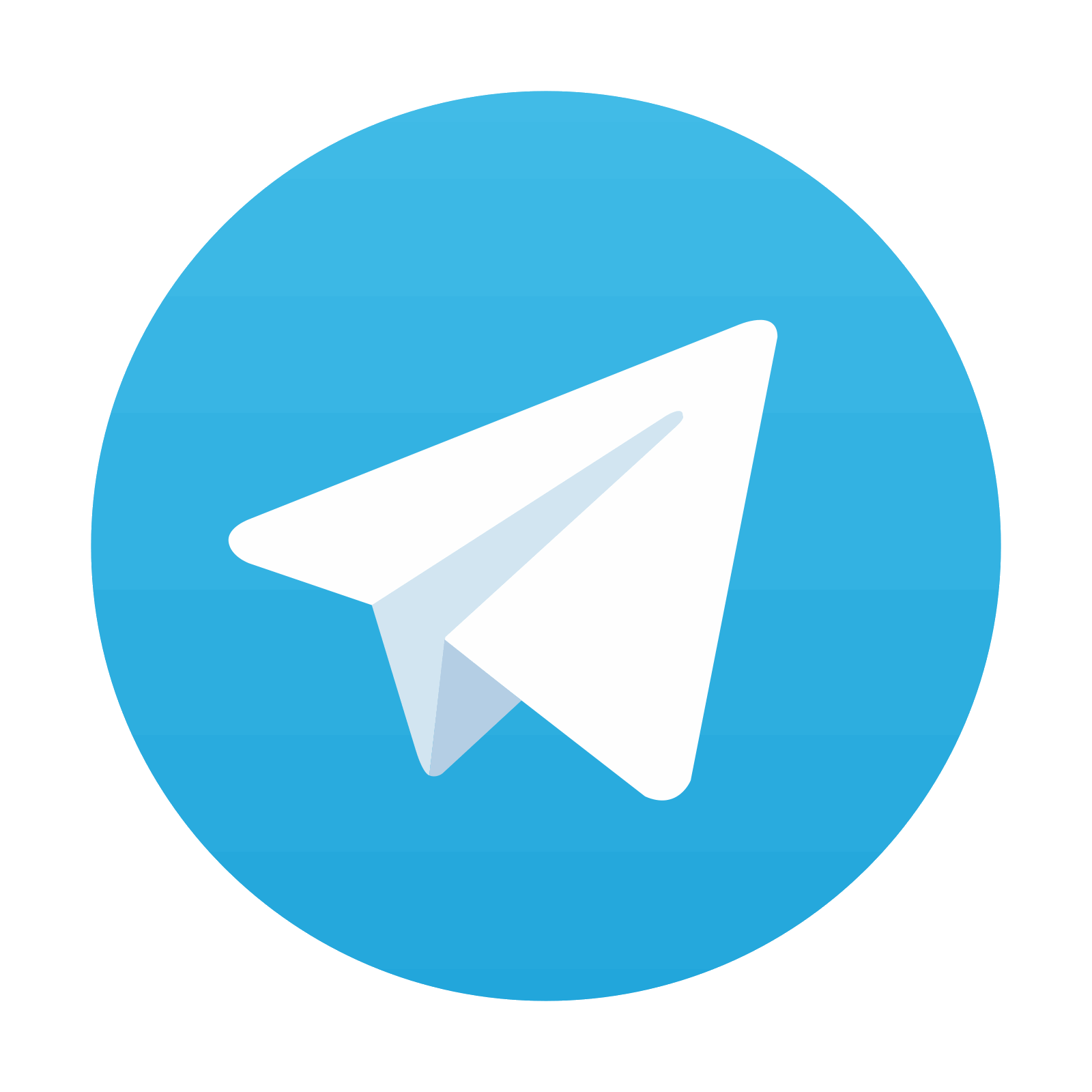
Stay updated, free articles. Join our Telegram channel
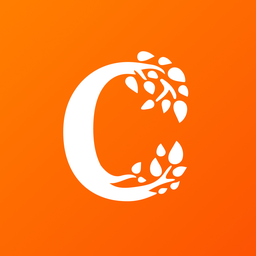
Full access? Get Clinical Tree
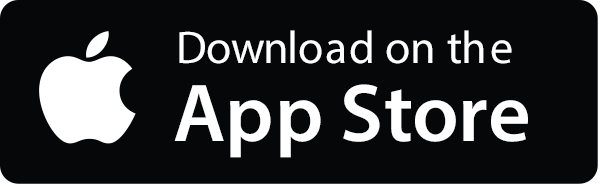
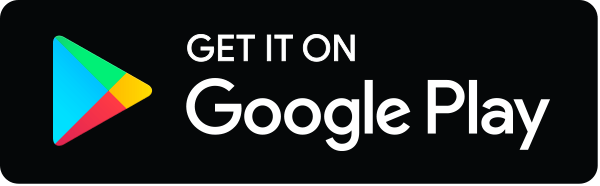