38
Thyroid & Antithyroid Drugs
CASE STUDY
A 33-year-old woman presents with complaints of fatigue, sluggishness, weight gain, cold intolerance, dry skin, and muscle weakness for the last 2 months. She is so tired that she has to take several naps during the day to complete her tasks. These complaints are new for her since she used to feel warm all the time, had boundless energy causing her some insomnia, and states she felt like her heart was going to jump out of her chest. She also states that she would like to become pregnant in the near future. Her past medical history is significant for radioactive iodine therapy (RAI) about 1 year ago after a short trial of methimazole and propranolol therapy. She underwent RAI due to her poor medication adherence and did not attend routine scheduled appointments afterward. On physical examination, her blood pressure is 130/89 mm Hg with a pulse of 50 bpm. Her weight is 136 lb (61.8 kg), an increase of 10 lb (4.5 kg) in the last year. Her thyroid gland is not palpable and her reflexes are delayed. Laboratory findings include a thyroid-stimulating hormone (TSH) level of 24.9 μIU/mL and a free thyroxine level of 8 pmol/L. Evaluate the management of her past history of hyperthyroidism. Identify the available treatment options for control of her current thyroid status.
THYROID PHYSIOLOGY
The normal thyroid gland secretes sufficient amounts of the thyroid hormones—triiodothyronine (T3) and tetraiodothyronine (T4, thyroxine)—to normalize growth and development, body temperature, and energy levels. These hormones contain 59% and 65% (respectively) of iodine as an essential part of the molecule. Calcitonin, the second type of thyroid hormone, is important in the regulation of calcium metabolism and is discussed in Chapter 42.
Iodide Metabolism
The recommended daily adult iodide (I−)* intake is 150 mcg (200 mcg during pregnancy and lactation).
Iodide, ingested from food, water, or medication, is rapidly absorbed and enters an extracellular fluid pool. The thyroid gland removes about 75 mcg a day from this pool for hormone synthesis, and the balance is excreted in the urine. If iodide intake is increased, the fractional iodine uptake by the thyroid is diminished.
Biosynthesis of Thyroid Hormones
Once taken up by the thyroid gland, iodide undergoes a series of enzymatic reactions that incorporate it into active thyroid hormone (Figure 38–1). The first step is the transport of iodide into the thyroid gland by an intrinsic follicle cell basement membrane protein called the sodium/iodide symporter (NIS). This can be inhibited by such anions as thiocyanate (SCN−), pertechnetate (TcO4−), and perchlorate (CIO4−). At the apical cell membrane a second I− transport enzyme called pendrin controls the flow of iodide across the membrane. Pendrin is also found in the cochlea of the inner ear. If pendrin is deficient or absent (PDS or SLC26A4 mutation), a hereditary syndrome of goiter and deafness, called Pendred’s syndrome, ensues. At the apical cell membrane, iodide is oxidized by thyroidal peroxidase (TPO) to iodine, in which form it rapidly iodinates tyrosine residues within the thyroglobulin molecule to form monoiodotyrosine (MIT) and diiodotyrosine (DIT). This process is called iodide organification. Thyroidal peroxidase is transiently blocked by high levels of intrathyroidal iodide and blocked more persistently by thioamide drugs. Gene expression of TPO is stimulated by thyroid-stimulating hormone (TSH).
FIGURE 38–1 Biosynthesis of thyroid hormones. The sites of action of various drugs that interfere with thyroid hormone biosynthesis are shown.
Two molecules of DIT combine within the thyroglobulin molecule to form L-thyroxine (T4). One molecule of MIT and one molecule of DIT combine to form T3. In addition to thyroglobulin, other proteins within the gland may be iodinated, but these iodoproteins do not have hormonal activity. Thyroxine, T3, MIT, and DIT are released from thyroglobulin by exocytosis and proteolysis of thyroglobulin at the apical colloid border. The MIT and DIT are then deiodinated within the gland, and the iodine is reutilized. This process of proteolysis is also blocked by high levels of intrathyroidal iodide. The ratio of T4 to T3 within thyroglobulin is approximately 5:1, so that most of the hormone released is thyroxine. Most of the T3 circulating in the blood is derived from peripheral metabolism of thyroxine (see below, Figure 38–2).
FIGURE 38–2 Peripheral metabolism of thyroxine. (Adapted, with permission, from Gardner DG, Shoback D [editors]: Greenspan’s Basic & Clinical Endocrinology, 8th ed. McGraw-Hill, 2007. Copyright © The McGraw-Hill Companies, Inc.)
Transport of Thyroid Hormones
Thyroxine and T3 in plasma are reversibly bound to protein, primarily thyroxine-binding globulin (TBG). Only about 0.04% of total T4 and 0.4% of T3 exist in the free form (as FT4 and FT3). Many physiologic and pathologic states and drugs affect T4, T3, and thyroid transport. However, the actual levels of free hormone generally remain normal, reflecting feedback control.
Peripheral Metabolism of Thyroid Hormones
The primary pathway for the peripheral metabolism of thyroxine is deiodination by three 5′deiodinase enzymes (D1, D2, D3). Deiodination of T4 may occur by monodeiodination of the outer ring, producing 3,5,3′-triiodothyronine (T3), which is three to four times more potent than T4. The D1 enzyme is responsible for most of the circulating T3 while D2 regulates T3 levels in the brain and pituitary. D3 deiodination produces metabolically inactive 3,3′,5′-triiodothyronine (reverse T3 [rT3]), (Figure 38–2). The low serum levels of T3 and rT3 in normal individuals are due to the high metabolic clearances of these two compounds.
Drugs such as amiodarone, iodinated contrast media, β blockers, and corticosteroids, and severe illness or starvation inhibit the 5′-deiodinase necessary for the conversion of T4 to T3, resulting in low T3 and high rT3 levels in the serum. A polymorphism in the D2 gene can reduce T3 activation and impair thyroid hormone response. The pharmacokinetics of thyroid hormones are listed in Table 38–1.
TABLE 38–1 Summary of thyroid hormone kinetics.
Evaluation of Thyroid Function
The tests used to evaluate thyroid function are listed in Table 38–2.
TABLE 38–2 Typical values for thyroid function tests.
A. Thyroid-Pituitary Relationships
Control of thyroid function via thyroid-pituitary feedback is also discussed in Chapter 37. Hypothalamic cells secrete thyrotropin-releasing hormone (TRH) (Figure 38–3). TRH is secreted into capillaries of the pituitary portal venous system, and in the pituitary gland, TRH stimulates the synthesis and release of thyrotropin (thyroid-stimulating hormone, TSH). TSH in turn stimulates an adenylyl cyclase–mediated mechanism in the thyroid cell to increase the synthesis and release of T4 and T3. These thyroid hormones act in a negative feedback fashion in the pituitary to block the action of TRH and in the hypothalamus to inhibit the synthesis and secretion of TRH. Other hormones or drugs may also affect the release of TRH or TSH.
FIGURE 38–3 The hypothalamic-pituitary-thyroid axis. Acute psychosis or prolonged exposure to cold may activate the axis. Hypothalamic thyroid-releasing hormone (TRH) stimulates pituitary thyroid-stimulating hormone (TSH) release, while somatostatin and dopamine inhibit it. TSH stimulates T4 and T3 synthesis and release from the thyroid, and they in turn inhibit both TRH and TSH synthesis and release. Small amounts of iodide are necessary for hormone production, but large amounts inhibit T3 and T4 production and release. Solid arrows, stimulatory influence; dashed arrows, inhibitory influence. H, hypothalamus; AP, anterior pituitary.
B. Autoregulation of the Thyroid Gland
The thyroid gland also regulates its uptake of iodide and thyroid hormone synthesis by intrathyroidal mechanisms that are independent of TSH. These mechanisms are primarily related to the level of iodine in the blood. Large doses of iodine inhibit iodide organification (Wolff-Chaikoff block, see Figure 38–1). In certain disease states (eg, Hashimoto’s thyroiditis), this can inhibit thyroid hormone synthesis and result in hypothyroidism. Hyperthyroidism can result from the loss of the Wolff-Chaikoff block in susceptible individuals (eg, multinodular goiter).
C. Abnormal Thyroid Stimulators
In Graves’ disease (see below), lymphocytes secrete a TSH receptor-stimulating antibody (TSH-R Ab [stim]), also known as thyroid-stimulating immunoglobulin (TSI). This immunoglobulin binds to the TSH receptor and stimulates the gland in the same fashion as TSH itself. The duration of its effect, however, is much longer than that of TSH. TSH receptors are also found in orbital fibrocytes, which may be stimulated by high levels of TSH-R Ab [stim] and can cause ophthalmopathy.
BASIC PHARMACOLOGY OF THYROID & ANTITHYROID DRUGS
THYROID HORMONES
Chemistry
The structural formulas of thyroxine and triiodothyronine as well as reverse triiodothyronine (rT3) are shown in Figure 38–2. All of these naturally occurring molecules are levo (L) isomers. The synthetic dextro (D) isomer of thyroxine, dextrothyroxine, has approximately 4% of the biologic activity of the L-isomer as evidenced by its lesser ability to suppress TSH secretion and correct hypothyroidism.
Pharmacokinetics
Thyroxine is absorbed best in the duodenum and ileum; absorption is modified by intraluminal factors such as food, drugs, gastric acidity, and intestinal flora. Oral bioavailability of current preparations of L-thyroxine averages 70% (Table 38–1). In contrast, T3 is almost completely absorbed (95%). T4 and T3 absorption appears not to be affected by mild hypothyroidism but may be impaired in severe myxedema with ileus. These factors are important in switching from oral to parenteral therapy. For parenteral use, the intravenous route is preferred for both hormones.
In patients with hyperthyroidism, the metabolic clearances of T4 and T3 are increased and the half-lives decreased; the opposite is true in patients with hypothyroidism. Drugs that induce hepatic microsomal enzymes (eg, rifampin, phenobarbital, carbamazepine, phenytoin, tyrosine kinase inhibitors, HIV protease inhibitors) increase the metabolism of both T4 and T3 (Table 38–3). Despite this change in clearance, the normal hormone concentration is maintained in the majority of euthyroid patients as a result of compensatory hyperfunction of the thyroid. However, patients dependent on T4 replacement medication may require increased dosages to maintain clinical effectiveness. A similar compensation occurs if binding sites are altered. If TBG sites are increased by pregnancy, estrogens, or oral contraceptives, there is an initial shift of hormone from the free to the bound state and a decrease in its rate of elimination until the normal free hormone concentration is restored. Thus, the concentration of total and bound hormone will increase, but the concentration of free hormone and the steady-state elimination will remain normal. The reverse occurs when thyroid binding sites are decreased.
TABLE 38–3 Drug effects and thyroid function.
Mechanism of Action
A model of thyroid hormone action is depicted in Figure 38–4, which shows the free forms of thyroid hormones, T4 and T3, dissociated from thyroid-binding proteins, entering the cell by the active transporters (eg, monocarboxylate transporter 8 [MCT8], MCT10, and organic anion transporting polypeptide [OATP1C1]). Transporter mutations can result in a clinical syndrome of mental retardation, myopathy, and low serum T4 levels (Allan-Herndon-Dudley syndrome). Within the cell, T4 is converted to T3 by 5′-deiodinase, and the T3 enters the nucleus, where T3 binds to a specific T3 thyroid receptor protein, a member of the c-erb oncogene family. (This family also includes the steroid hormone receptors and receptors for vitamins A and D.) The T3 receptor exists in two forms, α and β. Mutations in both α and β genes have been associated with generalized thyroid hormone resistance. Differing concentrations of receptor forms in different tissues may account for variations in T3 effect on these tissues.
FIGURE 38–4 Model of the interaction of T3 with the T3 receptor. A: Inactive phase—the unliganded T3 receptor dimer bound to the thyroid hormone response element (TRE) along with corepressors acts as a suppressor of gene transcription. B: Active phase—T3 and T4 circulate bound to thyroid-binding proteins (TBPs). The free hormones are transported into the cell by a specific transport system. Within the cytoplasm, T4 is converted to T3 by 5′-deiodinase (5′DI); T3 then moves into the nucleus. There it binds to the ligand-binding domain of the thyroid receptor (TR) monomer. This promotes disruption of the TR homodimer and heterodimerization with retinoid X receptor (RXR) on the TRE, displacement of corepressors, and binding of coactivators. The TR-coactivator complex activates gene transcription, which leads to alteration in protein synthesis and cellular phenotype. TR-LBD, T3 receptor ligand-binding domain; TR-DBD, T3 receptor DNA-binding domain; RXR-LBD, retinoid X receptor ligand-binding domain; RXR-DBD, retinoid X receptor DNA-binding domain; T3, triiodothyronine; T4, tetraiodothyronine, L-thyroxine. (Adapted, with permission, from Gardner DG, Shoback D [editors]: Greenspan’s Basic & Clinical Endocrinology, 8th ed. McGraw-Hill, 2007. Copyright © The McGraw-Hill Companies, Inc.)
Most of the effects of thyroid on metabolic processes appear to be mediated by activation of nuclear receptors that lead to increased formation of RNA and subsequent protein synthesis, eg, increased formation of Na+/K+-ATPase. This is consistent with the observation that the action of thyroid is manifested in vivo with a time lag of hours or days after its administration.
Large numbers of thyroid hormone receptors are found in the most hormone-responsive tissues (pituitary, liver, kidney, heart, skeletal muscle, lung, and intestine), while few receptor sites occur in hormone-unresponsive tissues (spleen, testes). The brain, which lacks an anabolic response to T3, contains an intermediate number of receptors. In congruence with their biologic potencies, the affinity of the receptor site for T4 is about ten times lower than that for T3. Under some conditions, the number of nuclear receptors may be altered to preserve body homeostasis. For example, starvation lowers both circulating T3 hormone and cellular T3 receptors.
Effects of Thyroid Hormones
The thyroid hormones are responsible for optimal growth, development, function, and maintenance of all body tissues. Excess or inadequate amounts result in the signs and symptoms of hyperthyroidism or hypothyroidism, respectively (Table 38–4). Since T3 and T4 are qualitatively similar, they may be considered as one hormone in the discussion that follows.
TABLE 38–4 Manifestations of thyrotoxicosis and hypothyroidism.
Thyroid hormone is critical for the development and functioning of nervous, skeletal, and reproductive tissues. Its effects depend on protein synthesis as well as potentiation of the secretion and action of growth hormone. Thyroid deprivation in early life results in irreversible mental retardation and dwarfism—typical of congenital cretinism.
Effects on growth and calorigenesis are accompanied by a pervasive influence on metabolism of drugs as well as carbohydrates, fats, proteins, and vitamins. Many of these changes are dependent upon or modified by activity of other hormones. Conversely, the secretion and degradation rates of virtually all other hormones, including catecholamines, cortisol, estrogens, testosterone, and insulin, are affected by thyroid status.
Many of the manifestations of thyroid hyperactivity resemble sympathetic nervous system overactivity (especially in the cardiovascular system), although catecholamine levels are not increased. Changes in catecholamine-stimulated adenylyl cyclase activity as measured by cAMP are found with changes in thyroid activity. Thyroid hormone increases the numbers of β receptors and enhances amplification of the β-receptor signal. Other clinical symptoms reminiscent of excessive epinephrine activity (and partially alleviated by adrenoceptor antagonists) include lid lag and retraction, tremor, excessive sweating, anxiety, and nervousness. The opposite constellation of effects is seen in hypothyroidism (Table 38–4).
Thyroid Preparations
See the Preparations Available section at the end of this chapter for a list of available preparations. These preparations may be synthetic (levothyroxine, liothyronine, liotrix) or of animal origin (desiccated thyroid).
Thyroid hormones are not effective and can be detrimental in the management of obesity, abnormal vaginal bleeding, or depression if thyroid hormone levels are normal. Anecdotal reports of a beneficial effect of T3 administered with antidepressants were not confirmed in a controlled study.
Synthetic levothyroxine is the preparation of choice for thyroid replacement and suppression therapy because of its stability, content uniformity, low cost, lack of allergenic foreign protein, easy laboratory measurement of serum levels, and long half-life (7 days), which permits once-daily to weekly administration. In addition, T4 is converted to T3 intracellularly; thus, administration of T4 produces both hormones and T3 administration is unnecessary. Generic levothyroxine preparations provide comparable efficacy and are more cost-effective than branded preparations, It is preferable that patients remain on a consistent levothyroxine preparation between refills to avoid changes in bioavailability. A branded soft gel capsule (Tirosint) had faster, more complete dissolution and was less affected by gastric pH or coffee than a tablet formulation.
Although liothyronine (T3) is three to four times more potent than levothyroxine, it is not recommended for routine replacement therapy because of its shorter half-life (24 hours), requiring multiple daily doses, and difficulty in monitoring its adequacy of replacement by conventional laboratory tests. T3 should also be avoided in patients with cardiac disease due to significant elevations in peak levels and a greater risk of cardiotoxicity. Using the more expensive thyroxine and liothyronine fixed-dose combination (liotrix) and desiccated thyroid has not been shown to be more effective than T4 administration alone. T3 is best reserved for short-term TSH suppression. Research is ongoing to clarify whether T3 might be more appropriate in patients with a polymorphism in the D2 gene who continue to report fatigue, weight gain, and mental impairment while on T4 alone.
The use of desiccated thyroid rather than synthetic preparations is never justified, since the disadvantages of protein antigenicity, product instability, variable hormone concentrations, and difficulty in laboratory monitoring far outweigh the advantage of lower cost. Significant amounts of T3 found in some thyroid extracts may produce significant elevations in T3 levels and toxicity. Equi-effective doses are 60 mg of desiccated thyroid, 88–100 mcg of levothyroxine, and approximately 37.5 mcg of liothyronine.
The shelf life of synthetic hormone preparations is about 2 years, particularly if they are stored in dark bottles to minimize spontaneous deiodination. The shelf life of desiccated thyroid is not known with certainty, but its potency is better preserved if it is kept dry.
ANTITHYROID AGENTS
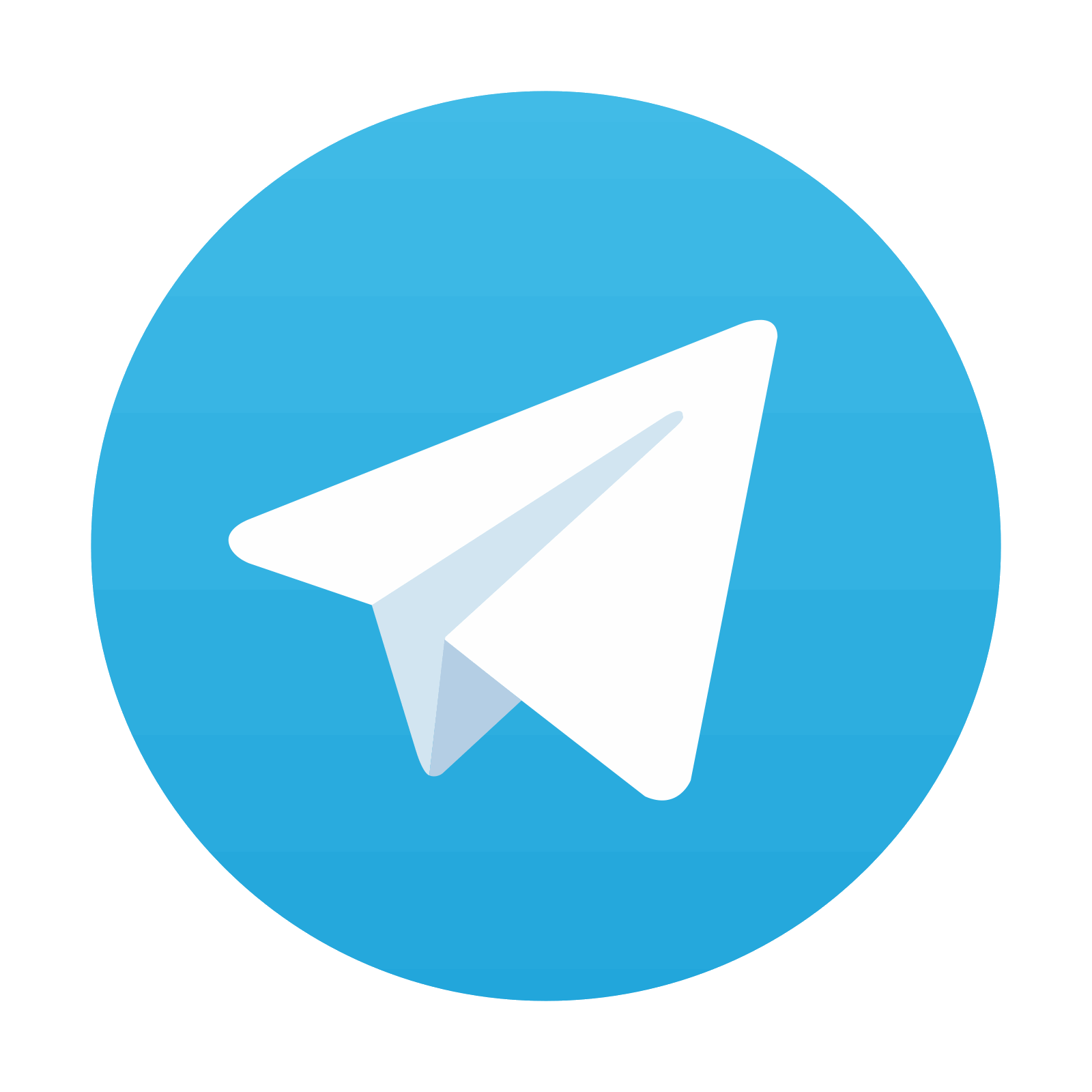
Stay updated, free articles. Join our Telegram channel
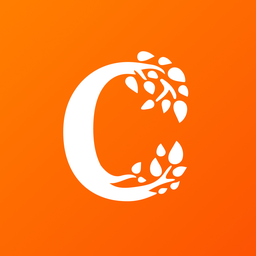
Full access? Get Clinical Tree
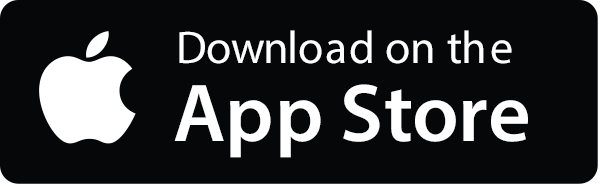
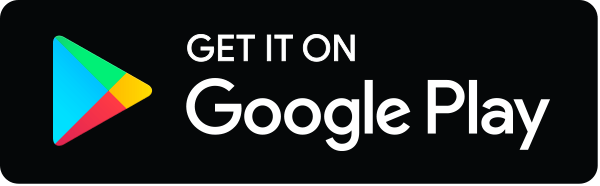