1 Introduction
There has long been a tendency, particularly in the medical and pharmaceutical fields, to regard microbes as harmful entities to be destroyed. However, as will be described in this chapter, the exploitation of microorganisms and their products has assumed an increasingly prominent role in the diagnosis, treatment and prevention of human diseases. Non-medical uses are also of significance, e.g. the use of bacterial spores (Bacillus thuringiensis) and viruses (baculoviruses) to control insect pests, the fungus Sclerotinia sclerotiorum to kill some common weeds, and improved varieties of Trichoderma harzianum to protect crops against fungal infections, and these will also be explored.
1.1 Early treatment of human disease
The earliest uses of microorganisms to treat human disease can be traced to the belief that the formation of pus in some way drained off noxious humours responsible for systemic diseases. Although the spontaneous appearance of pus in their patients’ wounds satisfied most physicians, deliberate contamination of wounds was also practiced. Bizarre concoctions of bacteria such as ‘ointment of pigs’ dung’ and ‘herb sclerata’ were particularly favoured during the Middle Ages. Early central European and South American civilizations cultivated various fungi for application to wounds. In the 19th century, sophisticated concepts of microbial antagonism were developed following Pasteur’ s experiments demonstrating inhibition of anthrax bacteria by ‘common bacteria’ simultaneously introduced into the same culture medium. Patients suffering with diseases such as diphtheria, tuberculosis and syphilis were treated by deliberate infection with what were then thought to be harmless bacteria such as staphylococci, Escherichia coli and lacto-bacilli. Following their discovery in the early part of the last century, bacterial viruses (bacteriophages) were considered as potential antibacterial agents—an idea that soon fell into disuse but has recently been revived (Chapter 27).
1.2 Present-day exploitation
Some of the most important and widespread uses of microorganisms in the pharmaceutical sciences are in the production of antibiotics and vaccines and the use of microorganisms in the recombinant DNA industry. These are described in Chapters 11, 24 and 25. However, there are a variety of other medicinal agents derived from microorganisms including vitamins, amino acids, dextrans, iron-chelating agents and enzymes. Microorganisms as whole or subcellular fractions, in suspension or immobilized in an inert matrix are employed in a variety of assays. Microorganisms have also been used in the pharmaceutical industry to achieve specific modifications of complex drug molecules such as steroids, in situations where synthetic routes are difficult and expensive to carry out, and more recently microorganisms have been employed in their own right as platforms for the discovery of novel therapeutic peptides and proteins.
2 Pharmaceuticals produced by microorganisms
2.1 Dextrans
Dextrans are polysaccharides produced by lactic acid bacteria, in particular members of the genus Leuconostoc (e.g. L. dextranicus and L. mesenteroides), following growth on sucrose. These sugar polymers first came to the attention of industrial microbiologists in sugar refineries where large gummy masses of dextran clogged pipelines. Dextran is essentially a glucose polymer consisting of (1–6)-α-links of high but variable molecular weight (15 000–20 000 000; Figure 26.1). Growth of the dextran producing strain is carried out in large fermenters using media with a low nitrogen but high carbohydrate content. The average molecular weight of the dextrans produced will vary with the strain used. This is important, since the laboratory or clinical utility of the dextran is dependent on a defined molecular weight. Two main methods are employed for obtaining dextrans of a suitable molecular weight. The first involves acid hydrolysis of very high molecular weight polymers, while the second uses small preformed dextrans as templates for the polymerization process. These templates are added to the culture fluid to produce dextrans of shorter chain length. Once formed, dextrans of the required molecular weight are obtained by precipitation with organic solvents prior to formulation.
Dextrans are produced commercially for use as plasma substitutes (plasma expanders), which can be administered by intravenous injection to maintain or restore the blood volume, and for application to ulcerated wounds or to burns where they form a hydrophilic layer that absorbs fluid exudates.
A summary of the properties of the different types of dextrans available is presented in Table 26.1. Dextrans for clinical use as plasma expanders require a molecular weight between 40 000 and 300 000. Polymers below 40 000 molecular weight are excreted too rapidly from the kidneys, while those above 300 000 molecular weight are potentially dangerous because of retention in the body. In practice, infusions containing dextrans of average molecular weights of 40 000, 70 000 and 110 000 are commonly encountered.
Table 26.1 Properties and uses of dextrans
IV, intravenous; IM, intramuscular.
The current British Pharmacopoeia and British National Formulary should be consulted for further information, including toxic manifestations.
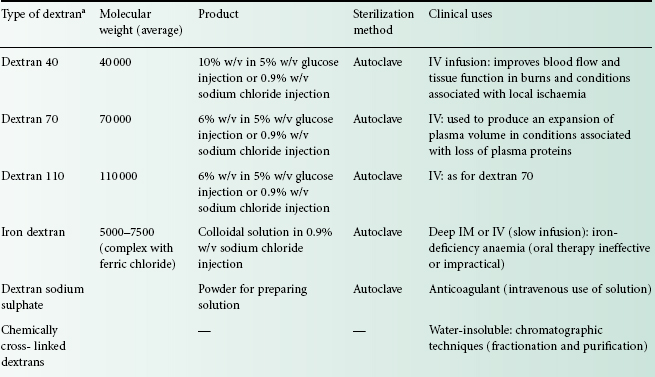
aIn the USA, dextran injections with average molecular weights of about 75 000 are also available.
Iron dextran injection, containing a complex of iron hydroxide with dextrans of average molecular weight between 5000 and 7000, is used for the treatment of iron-deficiency anaemia in situations where oral therapy is either ineffective or impractical. The sodium salt of sulphuric acid esters of dextran, i.e. dextran sodium sulphate, has anticoagulant properties comparable with those of heparin and is formulated as an injection for intravenous use.
2.2 Vitamins, amino acids and organic acids
Several chemicals used in medicinal products are produced by fermentation.
2.2.1 Vitamins
Vitamin B2 (riboflavin), one of the B group of vitamins, is present in milk, liver, kidneys, cereals and vegetables and is also synthesised by intestinal flora in carbohydrate-rich diets. Vitamin B2 deficiency, although rare, is characterized by symptoms that include an inflamed tongue, dermatitis and injury to the bone marrow. In genuine cases of malnutrition these symptoms will accompany those induced by other vitamin deficiencies. Riboflavin is produced commercially in significant yields by the moulds Eremothecium ashbyii and Ashbya gossypii and some bacteria including B. subtilis (Table 26.2).
Table 26.2 Examples of vitamins, amino acids, antibiotics and organic acids produced by microorganisms
Pharmaceutical | Producer organism | Use |
Riboflavin (vitamin B2) | Eremothecium ashbyii Ashbya gossypii | Treatment of vitamin B2 deficiency disease |
Cyanocobalamin (vitamin B12) | Propionibacterium freudenreichii Propionibacterium shermanii Pseudomonas denitrificans | Treatment of pernicious anaemia |
Amino acids, e.g., | Supplementation of feeds/food; intravenous infusion fluid constituents | |
Glutamate | Corynebacterium glutamicum | |
Lysine | Brevibacterium flavum | |
Antibiotics,a e.g. | Antibacterial drugs | |
Benzylpenicillin | Penicillium notatum, P. chrysogenum | |
Gentamicin | Micromonospora purpurea | |
Nystatin | Streptomyces noursei | |
Organic acids, e.g. | ||
Citric acid | Aspergillus niger | Effervescent products; sodium citrate used as an anticoagulant; potassium citrate used to treat cystitis |
Lactic acid | Lactobacillus delbrueckii Rhizopus oryzae | Calcium lactate is a convenient source of Ca2+for oral administration; constituent of intraperitoneal dialysis solutions |
Gluconic acid | Gluconobacter suboxydans Aspergillus niger | Calcium gluconate is a source of Ca2 + for oral administration; gluconates are used to render bases more soluble, e.g. chlorhexidine gluconate |
aFor further information, see Chapter 11.
Pernicious anaemia is a fatal disease first reported in 1880, but it was not until 1926 that it was discovered that eating raw liver effected a remission. The ‘anti-pernicious’ ingredient was subsequently isolated and called vitamin B12 or cyanocobalamin. Vitamin B12 was initially obtained from liver but during the 1960s it was determined that it could also be obtained as a by-product of microbial metabolism (Table 26.2). Hydroxycobalamin is the form of choice for therapeutic use and can be derived either by chemical transformation of cyanocobalamin or directly as a fermentation product.
Biotin, formerly known as vitamin H, is now regarded as another member of the vitamin B family and is found in similar food types. Biotin acts as an essential cofactor in chemical reactions that maintain normal metabolic function. It is also an essential growth factor for some bacteria. Its chemical structure was established in the early 1940s and a practical, highly stereospecific, chemical synthesis enabled d-biotin, identical to that found in yeasts and other cells, to be produced.
2.2.2 Amino acids
Amino acids find applications as ingredients of infusion solutions for parenteral nutrition and individually for the treatment of specific conditions. They are obtained either by fermentation processes similar to those used for antibiotics or in cell-free extracts employing enzymes isolated from bacteria (Table 26.2). Details of the many and varied processes reported in the literature will be found in the appropriate references in the ‘Further reading’ section at the end of the chapter.
2.2.3 Organic acids
Examples of organic acids (citric, lactic, gluconic) produced by microorganisms, together with pharmaceutical and medical uses, are given in Table 26.2. Citric and lactic acids also have widespread uses in the food and drink and plastics industries. Gluconic acid is also used as a metal-chelating agent in, for example, detergent products.
2.3 Iron-chelating agents
The growth of many microorganisms in iron-deficient growth media results in the secretion of low molecular weight iron-chelating agents called siderophores, which are usually phenolate or hydroxamate compounds. The therapeutic potential of these compounds has generated considerable interest. Uncomplicated iron deficiency can be treated with oral preparations of iron(II) (ferrous) sulphate but such treatment is not without hazard and iron salts remain a common cause of poisoning in children. The accidental consumption of around 3 g of ferrous sulphate by a small child leads to acidosis, coma and heart failure which, if untreated, are fatal. Desferrioxamine B (Figure 26.2), the deferrated form of a siderophore produced by Streptomyces pilosus, is a highly effective antidote for the treatment of acute iron poisoning. Desferrioxamine owes its effectiveness both to its high affinity for ferric iron (its binding constant is in excess of 10 30) and because the iron–desferrioxamine complex is highly water-soluble and is readily excreted through the kidneys. In haemolytic anaemias such as thalassaemia, desferrioxamine is used together with blood transfusions to maintain normal blood levels of free iron and haemoglobin. Desferrioxamine is prepared as a sterile powder for use as an injection, but it is also administered orally in acute iron poisoning to remove unabsorbed iron from the gut.
Patients with iron overload disorders treated with desferrioxamine may, however, have increased susceptibility to infections. The important role played by iron availability during infections in vertebrate hosts has only been recognized relatively recently. The ability of the host to withhold growth-essential iron from microbial and, indeed, neo-plastic invaders while retaining its own access to this metal has led to suggestions that microbial iron chelators or their semisynthetic derivatives may be of use in antimicrobial and anticancer chemotherapy. Preliminary work has shown some encouraging results. The bacterial siderophores parabactin and compound II secreted by Paracoccus denitrificans have been shown to inhibit the growth of leukaemia cells in culture and in experimental animals. They also appear capable of inhibiting the replication of RNA viruses. Siderophores such as desferrioxamine may therefore find increasing applications not only in the treatment of iron poisoning and iron-overloaded disease states but also as chemotherapeutic agents.
2.4 Enzymes
Several enzymes have important therapeutic, medical or pharmaceutical uses (Table 26.3). In this section, those enzymes used therapeutically will be described. Applications of microbially derived enzymes for antibiotic inactivation in sterility testing and diagnostic assays are discussed in section 5.
Table 26.3 Clinical uses and other applications of enzymes
AGAC, aminoglycoside-aminocyclitol antibiotics (see Chapter 11); CMP, chloramphenicol.
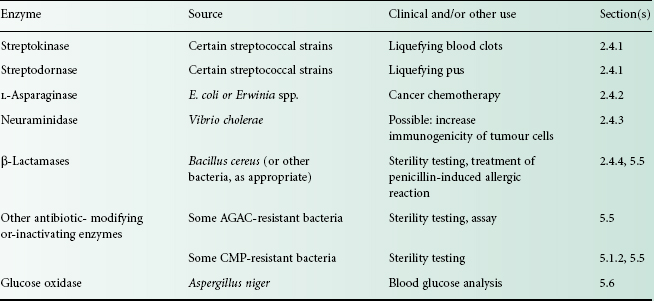
2.4.1 Streptokinase and streptodornase
Mammalian blood will clot spontaneously within minutes if allowed to stand, but if left to stand longer the clot begins to dissolve as a result of the action of a proteolytic enzyme called plasmin. Plasmin is normally present as its inactive precursor, plasminogen. Certain strains of streptococci were found to produce a substance that is capable of activating plasminogen (Figure 26.3), a phenomenon that suggested a potential use in liquefying clots, i.e. fibrinolysis. This substance, called streptokinase, was isolated and determined to be an enzyme.
Streptokinase is administered by intravenous or intra-arterial infusion in the treatment of thromboembolic disorders, e.g. pulmonary embolism, deep vein thrombosis and arterial occlusions. It is also used in emergency medicine for acute myocardial infarction.
A second enzyme, streptodornase, present in streptococcal culture filtrates, was observed to liquefy pus. Streptodornase is a deoxyribonuclease that breaks down deoxyribonucleoprotein and DNA, both constituents of pus, resulting in a reduction in pus viscosity. Streptokinase and streptodornase together have been used to facilitate drainage by liquefying blood clots and/or pus in the chest cavity. The combination can also be applied topically to wounds that have excessive suppuration.
Streptokinase and streptodornase are isolated following growth of non-pathogenic streptococcal producer strains in media containing excess glucose. They are obtained as a crude mixture from the culture filtrate and can be prepared relatively free of each other. They are commercially available as either streptokinase injection or as a combination of streptokinase and streptodornase.
2.4.2 L-Asparaginase
L-Asparaginase, an enzyme derived from E. coli or Erwinia chrysanthemi, converts l-asparagine to aspartic acid and ammonia. In contrast to normal tissue, some tumours have an essential requirement for l-asparagine and L-asparaginase has therefore been investigated as a selective cancer chemotherapeutic. Although L-asparaginase showed early promise in a variety of experimentally induced tumours, it has primarily found utility in humans for the treatment of acute lymphoblastic leukaemia and occasionally for myeloid leukaemia.
2.4.3 Neuraminidase
Many tumours escape immune surveillance through mechanisms including the masking of cell surface antigens by, for example, N-acetylneuraminic (sialic) acid residues. Neuraminidase, derived from Vibrio cholerae, has been used experimentally to increase the immunogenicity of tumour cells by stripping sialic acid residues from the outer surface of certain tumour cells resulting in presentation of tumour-specific antigens to the host immune system. In laboratory animals, administration of neuraminidase-treated tumour cells was found to be effective against a variety of mouse leukaemias. Preliminary investigations in acute myelocytic leukaemia patients have suggested that treatment of tumour cells with neuraminidase in combination with conventional chemotherapy may increase remission rates.
2.4.4 β-Lactamases
β-Lactamase enzymes, whilst presenting a considerable therapeutic challenge due to their ability to confer bacterial resistance by inactivating penicillins and cephalosporins (Chapter 13), are nevertheless useful in the sterility testing of certain antibiotics (see section 5.5) and, prior to culture, in inactivating various β-lactams in blood or urine samples in patients undergoing therapy with these drugs. One other important therapeutic application is in the rescue of patients presenting symptoms of a severe allergic reaction following administration of a β-lactamase-sensitive penicillin. In such cases, a highly purified penicillinase obtained from B. cereus has been administered either intramuscularly or intravenously and in combination with other supportive measures such as adrenaline and antihistamines.
3 Applications of microorganisms in the partial synthesis of pharmaceuticals
Microorganisms and microbially derived enzymes continue to play a significant role in the production of novel antibiotics. The potential of microorganisms as chemical catalysts, however, was a later development and first realized in the synthesis of industrially important steroids. These reactions assumed increasing importance following the discovery that certain steroids could be formulated as potent therapeutics, e.g. hydrocortisone has anti-inflammatory activity, and derivatives of the steroidal sex hormones are useful as oral contraceptive agents. More recently, chiral inversion of non-steroidal anti-inflammatory drugs (NSAIDs) has also been demonstrated.
3.1 Production of antibiotics
In the antibiotics industry, the hydrolysis of benzylpenicillin to give 6-aminopenicillanic acid by the enzyme penicillin acylase is an important stage in the synthesis of many clinically useful penicillins (Chapters 11). The combination of genetic engineering techniques to produce hybrid microorganisms with significantly higher acylase levels, together with their entrapment in gel matrices, which appears to improve the stability of the hybrids, has resulted in considerable increases in 6-aminopenicillanic acid yields.
A second example is provided by the production by fermentation of cephalosporin C, which is used solely for the subsequent preparation of semisynthetic cephalosporins (Chapter 11).
Furthermore, antibiotics produced by fermentation of various moulds and particularly Streptomyces spp., can be utilized by medicinal chemists as starting blocks in the production of what might be more effective antimicrobial compounds.
3.2 Steroid biotransformations
Previously, steroid hormones could only be obtained in small quantities directly from mammals and therefore attempts were made to synthesize them from plant sterols, which can be obtained economically in large quantities. However, adrenocortical steroids are characterized by the presence of an oxygen molecule at position 11 in the steroid nucleus and although it is relatively easy to hydroxylate a steroidal compound it is extremely difficult to achieve site-specific hydroxylation, such that many of the routes used for synthesizing the desired steroid are lengthy, complex and consequently expensive. This problem was overcome when it was realized that many microorganisms are capable of performing limited oxidations with both stereo-and regio-specificity. By simply adding a steroid to growing cultures of the appropriate microorganism, specific site-directed chemical changes can be introduced into the molecule. In 1952, the first commercially employed process involving the conversion of progesterone to 11 α-hydroxyprogesterone by the fungus Rhizopus nigricans was introduced (Figure 26.4). This reaction is an important stage in the manufacture of cortisone and hydrocortisone from more readily available steroids. Table 26.4 gives several other examples of microbially directed oxidations that have been or are employed in the manufacture of steroidal drugs.
Table 26.4 Examples of biological transformations of steroids
Starting material | Product | Type of reaction |
Progesterone | 11a-Hydroxyprogesterone | Hydroxylation |
Compound Sa | Hydrocortisone | Hydroxylation |
11α-Hydroxyprogesterone | D-11 α-Hydroxyprogesterone | Dehydrogenation |
Hydrocortisone | Prednisolone | Dehydrogenation |
Cortisone | Prednisone | Dehydrogenation |
aDerived from diosgenin by chemical transformation.
More recently, microorganisms utilized for biotrans-formation reactions have been immobilized by entrapment in a polymer gel matrix to avoid the often costly and time-consuming enzyme extraction steps that can result in enzyme inactivation. Immobilization also serves to increase the stability of membrane-associated enzymes that are unstable in the solubilized state, as well as permitting the conversion of water-insoluble compounds like steroids in two-phase water–organic solvent systems.
3.3 Chiral inversion
Several clinically relevant drugs including salbutamol (a β-adrenoceptor agonist), propanolol (a β-adrenoceptor antagonist) and the 2-arylpropionic acids (NSAIDs) are administered in their racemic form but undergo in vivo chiral inversion through metabolic transformations by microorganisms that mimic phase I metabolic processes, i.e. functionalization reactions. For example, the activity of NSAIDs (e.g. ibuprofen), resides almost exclusively in the S(+) isomers. However, unidirectional chiral inversion from R (–) to S(+) (Figure 26.5) occurs in vivo over a 3-hour period. The S(+) form is a more effective inhibitor of prostaglandin synthesis, and enzymes from some fungal species (e.g. Verticillium lecanii) convert a racemic mixture into the S(+) isomer in vitro. Another example is the biotransformation of ±propranolol to S(+) propranolol by R. arrhizus and Geotrichum candidum with around 83% efficiency.
4 Applications of microorganisms in the discovery of pharmaceuticals
Microbial display platforms expressing recombinant polypeptides (peptides, antibodies, enzymes) on their surface are emerging as invaluable tools for the investigation of protein–protein interactions and can serve as biological combinatorial libraries for the discovery of new therapeutics. To date three microbial display platforms have been described: phage, bacterial, and yeast display. All three technologies share the common principle of a direct link between genotype and phenotype affording the identification of the displayed polypeptide by gene sequencing. Of the three technologies described, it is probably phage display that has witnessed the most widespread application.
4.1 Phage display
The filamentous bacteriophage readily accepts relatively large insertions of additional genetic material into its genome, which allows for the display of polypeptides as fusions with bacteriophage coat proteins. Phage libraries containing a repertoire of many billions of viral particles that each display a unique polypeptide sequence can be subjected to an affinity selection process against a target of interest. Those phage clones that display a polypeptide that strongly interacts with the target can be recovered and amplified for further rounds of selection before the direct link between genotype and phenotype is exploited to identify the polypeptide displayed.
Although to date no phage-derived product has reached market, a number of clinical trials are currently under way using polypeptides derived from phage display: DX-88 (Ecallantide, Dyax Corp) is a highly specific and potent inhibitor of kallikrein (Ki ∼20–40 pM). Kallikrein is a key molecule in the regulation of infl ammatory and blood clotting processes and plays a role in a number of autoimmune and infl ammatory conditions. DX-88 has successfully completed phase III trials in the treatment of hereditary angiodema and phase I/II trials for the prevention of blood loss in on-pump coronary artery bypass graft surgery. Compstatin (Potentia Pharmaceutics), a cyclic 13-mer peptide originally identified from phage display, is a selective and potent inhibitor (Kd ∼0.13 μM) of C3 protein in the complement cascade and will shortly enter clinical trials for the treatment of age-related macular degeneration.
5 Use of microorganisms and their products in assays
Microorganisms have found widespread uses in bioassays for:
- determining the concentration of compounds (e.g. amino acids, vitamins and some antibiotics) in complex chemical mixtures or in body fluids
- diagnosing diseases
- testing chemicals for potential mutagenicity and carcinogenicity
- monitoring processes involving the use of immobilized enzymes
- sterility testing of antibiotics.
5.1 Antibiotic bioassays
Although antibiotics may be assayed by a variety of methods, the following section will only take into consideration microbiological and radioenzymatic assays.
5.1.1 Microbiological assays
In microbiological assays the response of a growing population of microorganisms to the antimicrobial agent under investigation is measured. The usual methods involve agar diffusion assays in which the drug diffuses into agar seeded with a susceptible microbial population producing a zone of growth inhibition.
In the commonest form of microbiological bioassay used today, samples to be assayed are applied in some form of reservoir (porcelain cup, paper disc or well) to a thin layer of agar seeded with indicator organism. The drug diffuses into the medium and after incubation a zone of growth inhibition forms, in this case as a circle around the reservoir. All other factors being constant, the diameter of the zone of inhibition is essentially related to the concentration of antibiotic in the reservoir.
During incubation the antibiotic diffuses from the reservoir and that part of the microbial population distant to the influence of the antibiotic increases by cell division. The edge of the area of microbial growth is formed when the minimum concentration of antibiotic that will inhibit the growth of the organism on the plate (critical concentration) reaches, for the first time, a population density too great for it to inhibit. The position of the zone edge is thus determined by the initial population density, the growth rate of the organism and the rate of diffusion of the antibiotic.
In situations where the likely concentration range of the tests will lie within a relatively narrow range (e.g. in determining potency of pharmaceutical preparations) and maximal precision is sought, then a Latin square design with tests and calibrators at two or three levels of concentration may be used. For example, an 8 × 8 Latin square can be used to assay three samples and one calibrator, or two samples and two calibrators at two concentrations each (over a twofold or fourfold range), with a coefficient of variation of around 3%. Using this technique, parallel dose–response lines should be obtained for the calibrators and the tests at the two dilutions (Figure 26.6). Using such a method, potency can be computed or determined from carefully prepared nomograms.
Figure 26.6 Graphical representation of a 2 × 2 assay response. X is the horizontal distance between the two lines. The antilog of X gives the relative potency of the standard and test.
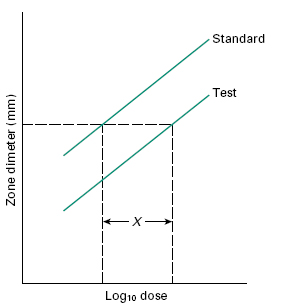
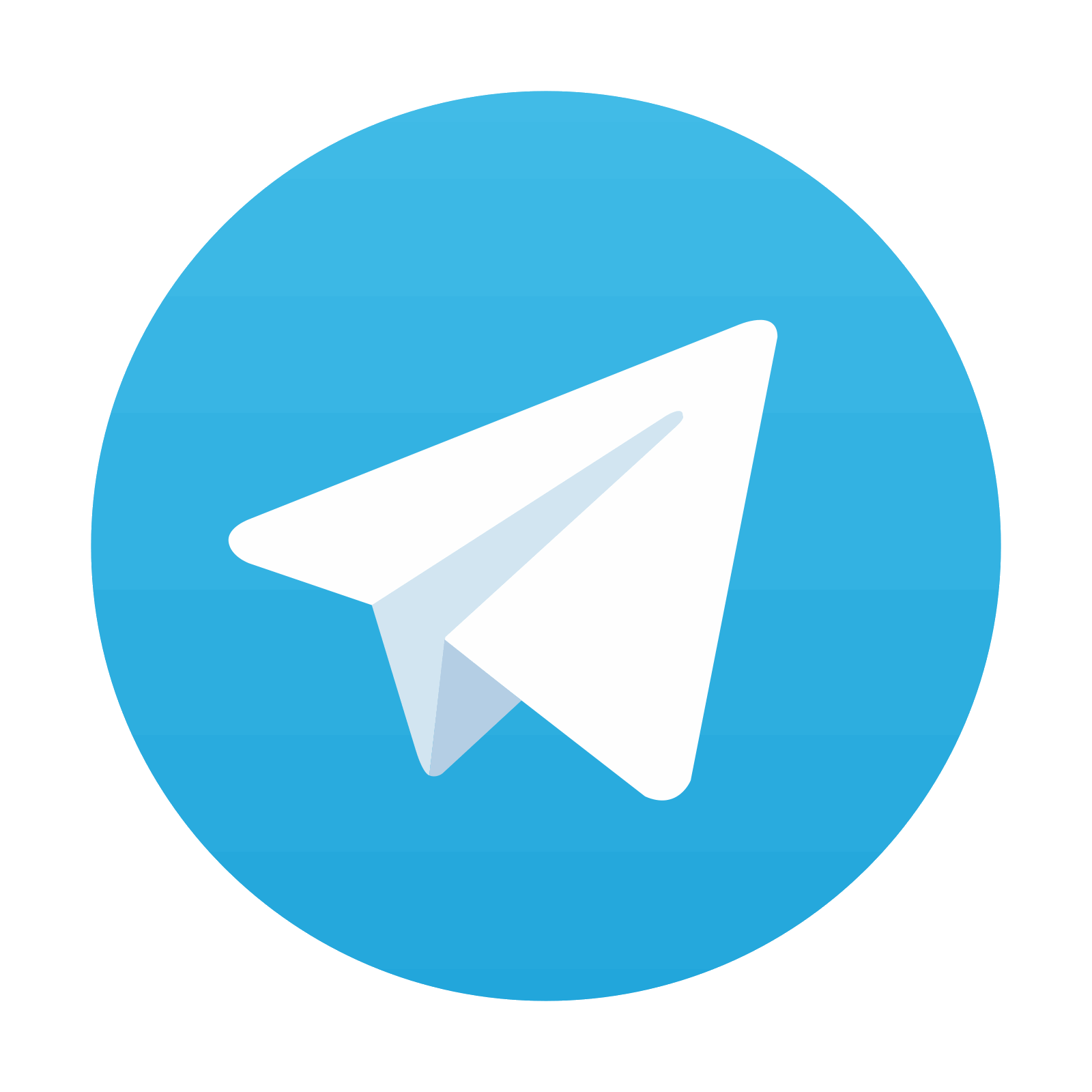