11 Respiratory system
ANATOMY
TRACHEA
BRONCHI
The trachea terminates at the level of the sternal angle by dividing into the right and left main bronchi (Fig. 11.1). The right main bronchus is wider, shorter and more vertical than the left. It is approximately 2.5 cm long and passes downwards and laterally behind the ascending aorta and SVC to enter the hilum of the lung. The azygos vein arches over it from behind to enter the SVC, while the pulmonary artery lies first below it and then anterior to it. The right main bronchus gives off an upper lobe bronchus just before it enters the lung. It then proceeds into the lung where it divides into the bronchi to the middle and inferior lobes.
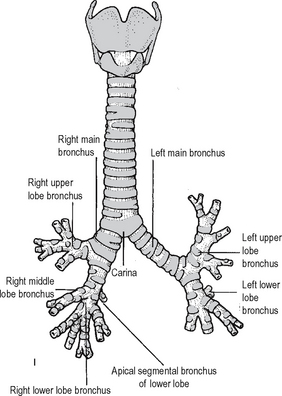
Fig. 11.1 The trachea and bronchi.
Source: Rogers A W Textbook of anatomy; Churchill Livingstone, Edinburgh (1992).
LUNGS
The lungs (Fig. 11.2) are conical in shape. They conform to the shape of the pleural cavities. Each lung has a blunt apex which reaches above the sternal end of the first rib, a base related to the diaphragm, a convex parietal surface related to the ribs, and a mediastinal surface which is concave and related to the pericardium.
Bronchopulmonary segments
Each lobar bronchus divides to supply the bronchopulmonary segments of the lung. Each lung has ten segments. These are shown in Fig. 11.3. Each of the bronchopulmonary segments is supplied by a segmental bronchus, artery and vein. There is no communication with adjacent segments. It is thus possible to remove an individual segment without interfering with the function of adjacent segments. Each segment takes its name from that of the supplying segmental bronchus.
PLEURA
Surface anatomy
The cervical pleura extends above the sternal end of the first rib. It follows a curved-line drawn from the sternoclavicular joint to the junction of the inner third and outer two-thirds of the clavicle, the apex of the pleura arising about 2.5 cm above the clavicle. The line of the pleura on each side passes from behind the sternoclavicular joint to meet in the midline at the level of the second costal cartilage. The right pleura then passes vertically down to the 6th costal cartilage before crossing the 8th rib in the midclavicular line, the 10th rib in the midaxillary line, and 12th rib at the lateral border of the erector spinae. On the left side the pleural edge reaches the 4th costal cartilage, where it arches out lateral to the border of the sternum, the pleura being separated from the chest wall by the protrusion of the pericardium. The medial ends of the 4th and 5th intercostal spaces are, therefore, notcovered by pleura. Apart from this, its relationshipsare the same as those on the right side. The pleura actually descends below the 12th rib at its medial extremity.
Clinical points
THORACIC CAGE
Ribs
There are 12 pairs of ribs (Fig. 11.4). The first seven pairs are connected anteriorly via their costal cartilages to the sternum. The 8th, 9th and 10th ribs articulate with their costal cartilages, each with the rib above. The 11th and 12th ribs remain free anteriorly and are known as ‘floating ribs’.
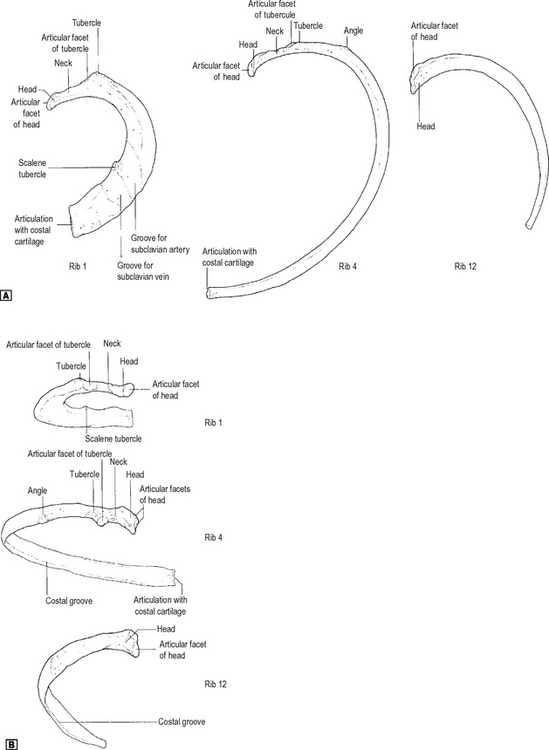
Fig. 11.4 Ribs 1, 4 and 12 viewed from the left side. superior view.
posterior view.
Source: Rogers op. cit.
The first, 2nd, 10th, 11th and 12th ribs are atypical. Only the first and 12th are clinically important. The first rib is the shortest, flattest and most curved of the ribs. It is flattened from above downwards. The features of the first rib are shown in Fig. 11.4. The 12th rib is short, has no tubercle, and has only a single facet. There is no angle and no subcostal groove. Its only importance is in the loin approach to the kidney, where its relationship to the pleura is important. The pleura descends below the 12th rib at its medial extremity.
Clinical points
Cervical ribs
These occur with an incidence of 1:200 and may be bilateral in 1:500 cases. The rib may be complete, articulating with the transverse process of the7th cervical vertebra behind and with the first rib in front. Occasionally, a cervical rib may have a free distal extremity and, in some cases, is merely represented bya fibrous band. A cervical rib may cause vascular or neurological symptoms. Vascular consequences include poststenotic dilatation of the subclavian artery, caused by local turbulence, and, therefore, the risk of distal emboli. A subclavian aneurysm may also result. It is also associated with Raynaud’s phenomenon. Pressure on the vein may result in subclavian vein thrombosis. A cervical rib may also cause pressure on the lower trunk of the brachial plexus which arches over it. This results in paraesthesia in the dermatomal distribution of C8/T1 together with wasting of the small muscles ofthe hand (myotome T1).
Sternum
This consists of three parts: the manubrium, the body and the xiphoid process.
Intercostal spaces
The neurovascular bundle runs between the internal intercostal and the innermost intercostal. It consists, from above downwards, of vein, artery and nerve, the vein lying directly in the groove on the undersurface of the corresponding rib. The arrangement of muscles, vessels and nerve is shown in Fig. 11.5.
DIAPHRAGM
The diaphragm (Fig. 11.6) is a dome-shaped septum separating the thorax from the abdomen. It is composed of a peripheral muscular part and a central tendon.
The muscular fibres arise from several sources: the crura, the arcuate ligaments, the ribs and the sternum. The right crus of the diaphragm arises from the front of the bodies of the first three lumbar vertebrae and the intervening intervertebral discs. The left crus arises from the first and second lumbar vertebrae and the intervening disc. The arcuate ligaments are a series of arches, the lateral being a condensation of the fascia overlying quadratus lumborum and the medial of the fascia overlying psoas major. The medial borders of the medial arcuate ligaments join anteriorly over the aorta as the median arcuate ligaments. The costal part of the diaphragm arises from the inner aspect of the lower six ribs and the sternal portion as two small slips from the posterior surface of the xiphisternum.
Nerve supply
The diaphragm is supplied by the phrenic nerves (C3, 4, 5) which have long course in the neck and the thorax. Damage to the nerve leads to paralysis of the diaphragm, which results in elevation of the diaphragm seen on x-ray and paradoxical movement on respiration. The phrenic nerve also gives a sensory supply to the central part of the diaphragm. Irritation of the diaphragm, e.g. in peritonitis or pleurisy, results in referred pain to the cutaneous area of supply, i.e. the shoulder tip via dermatome C4. The peripheral part of the diaphragm receives sensory innervation from the lower six intercostal nerves.
ANATOMY OF RESPIRATION
There are two main mechanisms for increasing the volume of the thorax:
Thoracic breathing
During inspiration, the ribs are elevated, and this occurs in two ways, as follows:
STRUCTURE OF THE RESPIRATORY TREE
The basic structure of the lower respiratory tree is shown in Fig. 11.7. The respiratory tree is designed to transport humidified air into the distal airways and alveoli where exchange between CO2 and oxygen takes place. The trachea is composed of C-shaped plates of cartilage with the curve of the C anteriorly; the ring is completed posteriorly with smooth muscle. The trachea contains mucous glands and is lined with ciliated epithelium. The trachea divides into bronchi and these contain discontinuous pieces of cartilage in their wall together with smooth muscle. They too are lined by columnar ciliated epithelium and contain mucous glands. The cilia beat rhythmically in a thin liquid layer and effectively transport the surface film of mucus and particles out of the lungs by way of the trachea.
MEDIASTINUM
The mediastinum (Fig. 11.8) is the name given to the space between the two pleural cavities. It extends from the sternum in front to the thoracic vertebrae behind and from the thoracic inlet above to the diaphragm below.
Posterior mediastinum
This lies behind the pericardium and the diaphragm below. Anteriorly lie the pericardium and roots of the lungs, with the diaphragm lying anteriorly below. Posteriorly lies the vertebral column from the lower border of the 4th to the 12th thoracic vertebrae. Inferiorly lies the diaphragm and superiorly is a horizontal plane drawn through the angle of Louis. The posterior mediastinum contains the descending thoracic aorta, the oesophagus, the vagus and splanchnic nerves, the azygos and hemiazygos veins, the thoracic duct and the posterior mediastinal lymph glands.
Because of the arrangement of structures in the mediastinum, its appearance is different when viewed from the right and left hand sides. These differences and their relationships to the roots of the lungs are shown in Figs. 11.9 and 11.10.
PHYSIOLOGY
CONTROL OF VENTILATION
The body succeeds in keeping arterial PO2 and PCO2 within remarkably narrow limits. This is made possible by highly developed negative feedback systems that consist of sensors, controllers and effectors. These are summarized in Table 11.1.
Table 11.1 Summary of the basic mechanisms controlling respiration
Controllers
Impulses from the brainstem effect normal automatic respiration:
Sensors
These are the central chemoreceptors, peripheral chemoreceptors, and several others. In the lung, sensors include primary stretch receptors, irritant receptors and J receptors. In the nose and upper airway there are sensitive irritant receptors, while joints and muscles utilise the gamma stretch receptor and associated reflex.
Receptors within the lung
How do these mechanisms combine to control ventilation?
Consider the three main chemical factors which affect respiration:
Arterial O2
In severe, longstanding, lung disease, patients may exhibit a persistent PaCO2 elevation with a low PaO2. These patients may rely on hypoxaemia to provide an adequate respiratory drive. If oxygen is administered to these patients it may well result in depression of ventilation. This is a relatively rare event and should not prevent the prescription of oxygen in adequate amounts to patients who remain tachypnoeic. It can be predicted by taking arterial blood for baseline gas analysis and then administering oxygen in increasing percentages. If the patient has a ventilatory drive dependent on hypoxaemia then the minute volume will decrease as oxygen is administered, and arterial carbon dioxide will increase. (NB: the response to hypoxaemia is mediated by peripheral chemoreceptors – it has no effect on central chemoreceptors except when hypoxia in the brain directly depresses output from the CNS.)
MECHANICS OF BREATHING
Elastic properties of the lung
As the thoracic volume increases during inspiration the lung tissues become stretched; the greater the degree of chest expansion, the greater the degree of stretching of the lungs. However this relationship is not entirely linear. Figure 11.11 shows the relationship between the volume of the lung and the negative pressure surrounding it. As the negative pressure increases, so the lung volume increases, up to a point where further negative pressure does not increase lung volume. When the pressure around the lung decreases, the lung volume also decreases, but it does not follow the same curve. This is called hysteresis. The lung volume at any given pressure during deflation is larger than that during inflation.
Because the change in lung volume per unit change in pressure will be larger in a large lung and smaller in a small lung, the compliance per unit volume of lung is often quoted. This is known as the specific compliance.
The following is a summary of the major factors affecting compliance:
Surface tension
Surface tension is defined as the force acting along an imaginary line drawn on the surface of a liquid. This force exists because of the strong cohesive forces between molecules along the surface. Its importance in the lung can be demonstrated by comparing the pressure volume behaviour in isolated lungs inflated with either water or air. Lungs inflated with air have a much greater compliance and so are easier to distend than lungs filled with water (Fig. 11.11).
Surfactant promotes several important properties within the lung, as follows:
Regional differences in ventilation
Closure of small airways
There is another important effect, which can be observed at low lung volumes. As the volume of the lung decreases during expiration the intrapleural pressure in the dependent regions becomes positive. The small airways begin to close, trapping gas within the distal alveoli. In normal subjects this airway closure only occurs at very low lung volumes. However in patients whose lungs have lost elastic tissue (for example, the elderly or those with emphysema), airway closure occurs at higher lung volumes. This airway closure can begin before the lung has reached its normal postexpiratory resting volume or functional residual capacity ((FRC) see later). The distal alveoli involved may be incompletely ventilated.
Sites of airway resistance
The major factors affecting airway resistance are the following.
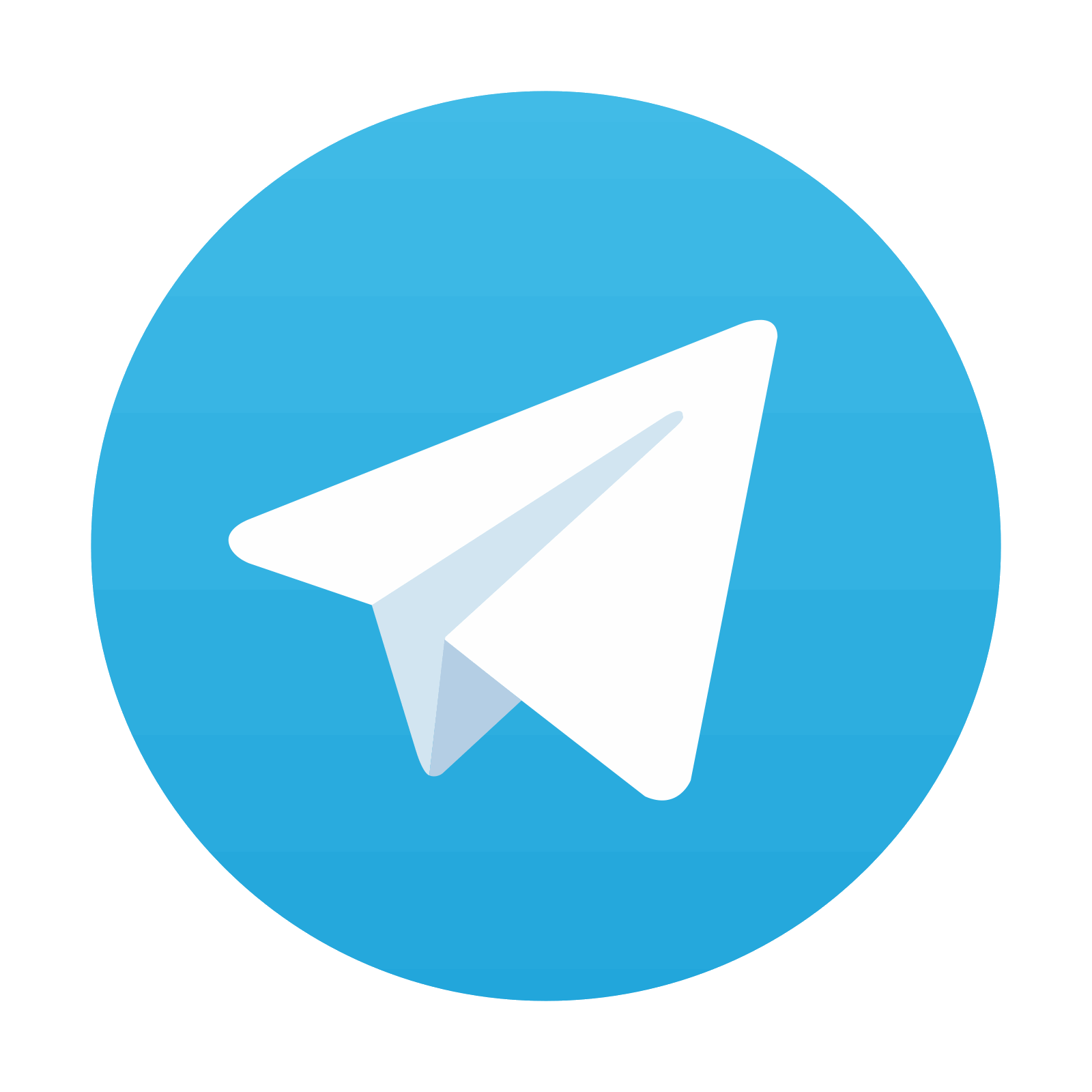
Stay updated, free articles. Join our Telegram channel
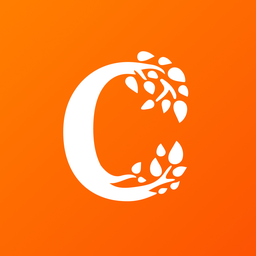
Full access? Get Clinical Tree
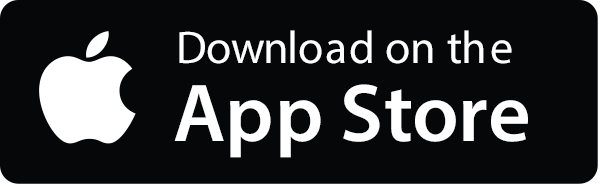
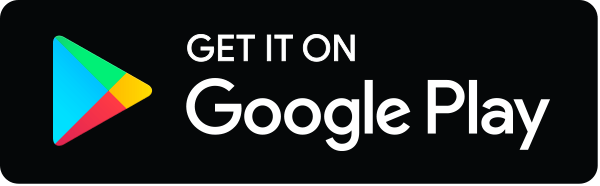