Scheme 7.1 The spectrum of uses for polymers in pharmaceutics
7.1.1 Definitions
Polymers are substances of high molecular weight made up of repeating monomer units. Substances with short chains containing relatively few monomers are called oligomers. Polymers owe their unique properties to their size, their three-dimensional shape and sometimes to their flexibility and asymmetry. The chemical reactivity of polymers depends on the chemistry of their monomer units, but their properties depend to a large extent on the way the monomers are assembled; it is this fact that leads to the versatility of synthetic polymers. Polymer molecules may be linear or branched, and separate linear or branched chains may be joined by crosslinks. Extensive crosslinking leads to a three-dimensional and often insoluble polymer network. Polymers in which all the monomeric units are identical are referred to as homopolymers; those formed from more than one monomer type are called copolymers. Various arrangements of the monomers A and B in the copolymer molecules (Fig. 7.1) can be produced with consequent effects on the physical properties of the resulting polymer. Synthetic polymers may have their main chains substituted in different ways, depending on the conditions of the reaction, such that atactic (random), isotactic or syndiotactic forms are produced, as diagrammatically represented in Fig. 7.1.
Figure 7.1 (a) Varieties of copolymer molecular structures attainable though the polymerisation of two different monomers represented by A and B. (b) Representations of random atactic, isotactic and symmetrical copolymers.
Copolymers may be described as alternating copolymers, block copolymers or graft copolymers. The molecular architecture of copolymers may, however, be more complicated than represented in Fig. 7.1. Block copolymers such as the polyoxyethylene–polyoxypropylene–polyoxyethylene (ABA) systems have both hydrophobic (A) and hydrophilic regions (B); they form an interesting group of surfactants and are discussed in Chapter 5. Homopolymers can be linear, star or branched (Fig. 7.2), giving rise to so-called star block copolymers defined by the number of arms (n).
Figure 7.2 The range of structures of homopolymers and star block, graft block and multiblock copolymers.
Polymers that have fairly symmetrical chains and strong interchain forces can be drawn into fibres. Plastics are polymers with lower degrees of crystallinity that can be moulded. Further down the rigidity scale are rubbers and elastomers, whose properties are well known.
It is apparent that polymer molecules will have a much wider range of physical properties than small chemical entities. Even when considering one chemical type (for example, polyethylene), its properties may be altered by increasing or decreasing the molecular weight (Fig. 7.3). There is a degree of control over properties that is not present with small organic materials. It is because of this that synthetic polymers also have an advantage over many variable natural polymers, although many natural derivatives such as cellulosic derivatives are used extensively. Natural materials can be modified chemically, and this approach can lead to useful new products, as with those derived from cellulose or dextran. The structural formulae of some common macromolecules are given in Table 7.1.
Figure 7.3 Approximate relation between molecular weight, glass transition temperature (Tg), melting point (Tm) and polymer properties.
Reproduced from Billmeyer FW. Textbook of Polymer Science, 2nd edn. New York: Wiley; 1971.
Table 7.1 Structural formulae of some macromolecular compounds
Name | Chain structure | Monomer |
Polymers with a carbon chain backbone | ||
Polyethylene | ||
Polypropylene | ||
Polystyrene | ||
Poly(vinyl chloride) | ||
Polytetrafluoroethylene | ||
Polyacrylonitrile | ||
Poly(vinyl alcohol) | ||
Poly(vinyl acetate) | ||
Polyacrylamide | ||
Poly(methyl methacrylate) | ||
Polyvinylpyrrolidone | ||
Polymers with a heterochain backbone | ||
Poly(ethylene oxide) | ||
Poly(propylene oxide) | ||
Cellulose (polyglucoside, β → 1,4) | ||
Chitosan |
| |
Amylose (polyglucoside, α → 1,4) (component of starch) | Glucose | |
Pectinic acid (polygalacturonoside, α → 1,4) (jelly-forming component of fruits) | ||
Polyethylene glycol terephthalate |
| |
Polydimethylsiloxane |
|
Dendrimers are highly branched polymer constructs formed from a central core that defines their initial geometry.1 Their branch-like structure (Fig. 7.4) leads to spheres, which in higher generations appear to be the size of micelles, and ultimately nanospheres of small dimensions. They can be functionalised and in this way ‘layered’ systems can be formed by using different monomers for succeeding reactions (generations); such chemical architecture has virtually no bounds.2 Dendrons are partial dendrimers, usually having a branched structure coupled to a linear chain.
Figure 7.4 (a) A two-dimensional view of a dendrimer with 64 cyano functional groups. It is possible to trap small to medium-size molecules in dendrimers that have pores of appropriate dimensions between the branches of the structure. (b) A diagrammatic representation of possible sites for covalent attachment of drugs, solubilising groups and targeting moieties, and encapsulation of drugs.
(a) Reprinted by permission from Macmillan Publishers Ltd: Nature. Gibson HW. Architectural delights. Nature 1994;371:106, copyright 1994.
7.1.2 Polydispersity
Nearly all synthetic polymers, possibly with the exception of dendrimers and some low-molecular-weight systems, and naturally occurring macromolecular substances exist with a range of molecular weights; exceptions to this are proteins and natural polypeptides, each of which occurs with a single well-defined molecular weight. The molecular weight of a polymer or macromolecule is thus an average molecular weight, which may be determined by chemical analysis or by osmotic pressure or light-scattering measurements. When determined by chemical analysis or osmotic pressure measurement a number-average molecular weight, Mn, is found, which in a mixture containing n1, n2, n3, … moles of polymer with molecular weights M1, M2, M3, …, respectively, is defined by
The individual molecular weights M1, M2, … cannot be determined separately – the equation merely explains the meaning of the value Mn.
In light-scattering techniques, larger molecules produce greater scattering; thus the weight (or, more strictly, the mass) rather than the number of the molecules is important, giving a weight-average molecular weight, Mw:
In equation (7.2) m1, m2, m3, … are the masses of each species, and mi is obtained by multiplying the molecular weight of each species by the number of molecules of that weight; that is, mi = niMi. Thus the molecular weight appears as the square in the numerator of equation (7.2); the weight-average molecular weight is therefore biased towards larger molecules. Another consequence is that Mw > Mn; that is, the average molecular weight of a polymer measured by light scattering must be greater than that obtained by osmotic pressure measurements if the polymer is polydisperse (that is, contains a range of molecular weights). The ratio Mw/Mn expresses the degree of polydispersity. Table 7.2 shows actual values for the number-average and weight-average molecular weights for dextrans, microbial polysaccharides used therapeutically as plasma expanders.
Table 7.2 Number- and weight-average molecular weights for dextran fractions
Fraction | Mn | Mw | Mw/Mn |
A | 41 000 | 47 000 | 1.14 |
B | 38 000 | 50 000 | 1.31 |
C | 64 000 | 76 000 | 1.18 |
D | 95 000 | 170 000 | 1.79 |
E | 240 000 | 540 000 | 2.25 |
Reproduced from Wales M et al. Intrinsic viscosity-molecular weight relationships for dextran. J Polymer Sci 1953;10:229. Copyright Wiley-VCH Verlag GmbH & Co. KGaA. Reproduced with permission.
7.1.3 Polymer mixtures or blends
Of course not all polymers are soluble or miscible with each other; some indeed de-mix when added together. The different phase behaviours that are realised with representative molecular architectures are shown in Fig. 7.5. Such mixtures produce solid polymeric structures such as films and membranes with distinctive morphologies and properties. When polymer blends are used as drug delivery matrices or as the basis of micro- and nanoparticles, it is important that polymer–polymer interactions are studied.
Figure 7.5 Representative polymer–polymer phase behaviour with different molecular architectures. Microphase separation (a) results when thermodynamically incompatible linear homopolymers are mixed. The covalent bond between blocks in a diblock copolymer leads to microphase segregation (c). A mixed architecture of linear homopolymers and the corresponding diblock copolymer produces a surfactant-like stabilised intermediate-scale phase separation (b).
7.1.4 Polymer solubility
The solubility of polymeric substances in water is determined by the same considerations that apply to smaller molecules. Those polymers that are sufficiently polar will be able to interact with the water to provide energy to remove individual polymer chains from the solid state.
Water-soluble polymers have an ability to increase the viscosity of solvents at low concentrations, to swell or change shape in solution, and to adsorb at surfaces. These are significant features of their behaviour, which we will deal with briefly.
Insoluble polymers or polymers with a low rate of solution are used more to form thin films, as film-coating materials, surgical dressings or membranes for dialysis or filtration, or to form matrices for enveloping drugs to control their release properties, or simply as packaging materials.
Figure 7.6 illustrates the variety of morphologies that polymeric systems can adopt depending on the nature of the solvent, the polymer concentration and the nature of the polymer itself. Such diversity explains the wide range of uses in pharmacy and medicine.
Figure 7.6. Representation of the variety of polymer morphologies in solution and in the gel (or microgel) or solid states. In solution the conformation of the polymer depends on the nature of polymer–solvent interactions and whether or not the polymer chains associate to form micellar aggregates. Crystals of polymer and microcrystals can be prepared, and gels can be formed from covalently crosslinked chains or polymer chains associated by hydrogen bonding or hydrophobic interactions. Listed are the forms in which most polymers can be fabricated: membranes, fibres, composites, matrices; microspheres and microcapsules can also feature, as discussed later in this chapter.
|
|
7.2 Water-soluble polymers
The rate of solution of a water-soluble polymer depends on its molecular weight: the larger the molecule, the stronger are the forces holding the chains together. More energy has to be expended to force the chains apart in the solvent. The greater the degree of crystallinity of the polymer, the lower the rate of solution.
The velocity of penetration (S) of a solvent into the bulk polymer obeys the relationship
where M is the polymer molecular weight, k and A being constants. The dissolution process, however, is more complicated than with ordinary crystalline materials. It is frequently observed that swollen layers and gel layers form next to the polymer (Fig. 7.7). If a drug is embedded in the polymer, the drug has to diffuse through these gel layers and finally through the diffusion layer.
Figure 7.7 Penetration of solvent into (top) soluble crystalline material and (bottom) polymer compared.
It is the combination of slow solution rate and the formation of viscous surface layers that makes hydrophilic polymers useful in controlling the release rate of soluble drugs (see section 7.5). Choice of appropriate polymer molecular weight controls both the rate of dissolution and the viscosity of its resulting solution. A balance between rate of polymer solution and viscosity of the solution layer must be achieved in controlled-release systems. If the polymer solution rate is too slow, then soluble drug is leached out with little retardation.
The bulk viscosity of polymer solution is an important parameter also when polymers are being used as suspending agents to maintain solid particles in suspension by prevention of settling (see Chapter 6) and when they are used to modify the properties of liquid medicines for oral and topical use.
7.3 General properties of polymer solutions
7.3.1 Viscosity of polymer solutions
The rheology of colloidal solutions and suspensions has been discussed in Chapter 6. Here we consider the particular use of viscosity measurements to determine the average molecular weights and asymmetry of polymeric molecules.
The presence in solution of large macromolecular solutes has an appreciable effect on the viscosity of the solution. From a study of the viscosity as a function of polymer concentration it is possible to gain information on the shape or hydration of these polymers in solution and also their average molecular weight. The assumption is made in this section that the solution exhibits Newtonian flow characteristics.
As discussed in Chapter 6 (section 6.3), the viscosity of solutions of macromolecules is conveniently expressed by the relative viscosity, rel, defined as the ratio of the viscosity of the solution,
, to the viscosity of the pure solvent
0; the specific viscosity,
sp, defined as
rel – 1; and the reduced viscosity
sp/
(where
is the volume fraction, defined as the volume of the particles divided by the total volume of the solution). The ratio
sp/
for ideal solutions is independent of solution concentration. In real solutions the reduced viscosity varies with concentration owing to molecular interactions so it is usual to extrapolate plots of
sp/
versus
to zero volume fraction. The extrapolated value is called the intrinsic viscosity [
].
Einstein showed from hydrodynamic theory that for a dilute system of rigid, spherical particles
i.e.
where is the volume fraction of the particles, defined as the volume of the particles divided by the total volume of the solution. In other words, the intrinsic viscosity [
] of this ideal system is 2.5.
For polymers of high molecular weight, the value of sp/
varies with solution concentration (which is directly related to
). The concentration dependence is often expressed as
where k is a constant referred to as the Huggins’ constant. At moderate concentrations a plot of sp/c against c is linear with a gradient equal to k. The value of this constant provides a useful indication of the extent of interaction between the polymer and the solvent; for flexible polymer molecules in a ‘good’ solvent (see below) k often has a value of approximately 0.35.
Departure of the limiting value of sp/
from the theoretical value of 2.5 may result from either hydration of the particles, or from particle asymmetry, or from both.
A more general form of equation (7.4) allowing for particle asymmetry is
where the intrinsic viscosity [] is replaced by a shape factor ν related to the axial ratio of an ellipsoid. In the case of non-hydrated spheres ν reduces to 2.5.
The volume fraction is usually replaced by the weight concentration, c. For a macromolecule of hydrodynamic volume vh and molecular weight M, =c NAvh/M where NA is the Avogadro constant.
Macromolecules are frequently hydrated, not only with chemically bound water but also with physically entrapped solvent. These two types of water have differing properties; the specific volume v0 (volume per gram) of the entrapped water may be considerably different from that of pure solvent, . The intrinsic viscosity of the hydrated macromolecule may be written as
where 1 is the number of grams of solvent per gram of dry macromolecular material and
is its partial specific volume.
If the particle can be assumed to be unhydrated, or if the degree of hydration can be estimated with certainty from other experimental techniques, equation (7.8) may be used to determine the asymmetry of the particle. Alternatively, if the macromolecule may be assumed to be symmetrical or its asymmetry is known from other techniques, then this equation may be used to estimate the extent of hydration of the macromolecule.
Shape and solvent effects
As the shape of molecules is to a large extent the determinant of flow properties, change in shape due to changes in polymer–solvent interactions and the binding of small molecules with the polymer may lead to significant changes in solution viscosity. The nature of the solvent is thus of prime importance in this regard. In so-called ‘good’ solvents, linear macromolecules will be expanded as the polar groups will be solvated. In a ‘poor’ solvent, the intramolecular attraction between the segments is greater than the segment–solvent affinity and the molecule will tend to coil up (Fig. 7.6). The viscosity of ionised macromolecules is complicated by charge interactions that vary with polymer concentration and additive concentration. Flexible charged macromolecules will vary in shape with the degree of ionisation. At maximum ionisation they are stretched out owing to mutual charge repulsion and the viscosity increases. On addition of small counterions, the effective charge is reduced and the molecules contract; the viscosity falls as a result. Some of the effects are illustrated later in this chapter in discussion of individual macromolecules.
The viscosity of solutions of globular proteins (which are more or less rigid) is only slightly affected by change in ionic strength. The intrinsic viscosity of serum albumin varies only between 3.6 and 4.1 cm3 g−1 when the pH is varied between 4.3 and 10.5 and the ionic strength between zero and 0.50.
Viscosity in pharmacopoeial specifications
In cases where control of molecular weight is important, for example in the use of dextran fractions as plasma expanders,3 a viscosity method is specified, for example, in the BP monograph. Staudinger proposed that the reduced viscosity of solutions of linear high polymers is proportional to the molecular weight of the polymer or its degree of polymerisation, p:
This empirical law has been modified to
where a is a constant in the range 0–2, which for most high polymers has a value between 0.6 and 0.8, [] is the intrinsic viscosity as defined previously, and M is the molecular weight of the polymer. Equation (7.10) is often referred to as the Mark–Houwink equation. For a given polymer–solvent system, K and a are constant. Values of these constants may be determined from measurements on a series of fractions of known molecular weight and hence the molecular weight of an unknown fraction can be determined by measurement of the intrinsic viscosity. The viscosity-average molecular weight is essentially a weight-average since the larger macromolecules influence viscosity more than the smaller ones. The intrinsic viscosity of Dextran 40 BP is stated to be not less than 16 cm3 g−1 and not more than 20 cm3 g−1 at 37°C, while that of Dextran 110 is not less than 27 cm3 g−1 and not more than 32 cm3 g−1.
|
the relative viscosity, the specific viscosity, the intrinsic viscosity [
|
7.3.2 Gel formation
Concentrated polymer solutions frequently exhibit a very high viscosity because of the interaction of polymer chains in a three-dimensional fashion in the bulk solvent. These viscous crosslinked systems are termed gels. A gel is a polymer-solvent system containing a three-dimensional network of quite stable bonds that are almost unaffected by thermal motion. If such a polymer network is surrounded by the solvent (the system can be arrived at by swelling of solid polymer or by reduction in the solubility of the polymer in the solution) the system is a gel regardless of whether the network is formed by chemical or physical bonds. When gels are formed from solutions, each system is characterised by a critical concentration of gelation below which a gel is not formed. This concentration is determined by the hydrophile–lipophile balance of the polymer and the degree of regularity of the structure, by polymer–solvent interaction, by molecular weight and by the flexibility of the chain: the more flexible the molecule, the higher is the critical gelling concentration. The characteristic features of a gel include the considerable increase in viscosity above the gel point, the appearance of a rubber-like elasticity and, at higher polymer concentrations, a yield point stress. Under small stress the gel should retain its shape, but considerable deformation can occur at higher stress.
Type I and type II gels
Gels can be categorised into two groups, depending on the nature of the bonds between the chains of the network. Gels of type I are irreversible systems with a three-dimensional network formed by covalent bonds between the macromolecules. They include swollen networks, which have been formed by polymerisation of a monomer in the presence of a crosslinking agent.
Type II gels are heat-reversible, being held together by intermolecular bonds such as hydrogen bonds. Sometimes bridging by additive molecules can take place in these type II systems. Poly(vinyl alcohol) solutions gel on cooling below a temperature known as the gel point. The gel point can therefore be influenced by the presence of additives that can induce gel formation by acting as bridge molecules, as, for example, with borax and poly(vinyl alcohol). The gel point of polymers can also be increased or decreased by the addition of solvents that alter the polymer’s affinity for the solvent (Table 7.3).
Table 7.3 Gel points of 10% poly(vinyl alcohol)
Solvent | Gel point (°C) |
Water | 14 |
Glycerol | 64 |
Ethylene glycol | 102 |
Reproduced from Pritchard JG. Poly(vinyl alcohol): Basic Properties and Uses. London: Macdonald; 1970.
Solutions of vinyl alcohol polymers in water are viscous mucilages that resemble those formed by methylcellulose; the viscosity of the mucilage is greatly increased by incorporating sodium perborate or silicate. Because of their gelling properties poly(vinyl alcohol) solutions are used as jellies for application of drugs to the skin. On application, the gel dries rapidly, leaving a plastic film with the drug in intimate contact with the skin. Plastic Film (Canadian Pharmacopoeia) is prepared from poly(vinyl alcohol) and other additives and is intended as a vehicle for acriflavine, benzocaine, ichthammol and other topical drugs.
Depending on the polymer, gelation can occur either with a fall or a rise in the temperature.
Crosslinked polymeric systems
If water-soluble polymer chains are covalently crosslinked, gels will be formed when the dry material interacts with water. The polymer swells in water but cannot dissolve as the crosslinks are stable. This expansion on contact with water has been put to many uses, such as in the fabrication of expanding implants from crosslinked hydrophilic polymers that imbibe body fluids and swell to a predetermined volume. These materials, such as the poly(hydroxyethyl methacrylate)s (poly(HEMA)s), are insoluble and chemically stable because of their three-dimensional structure (I and II) and do not dissolve. Implanted in the dehydrated state, these polymers swell to fill a body cavity or to give form to surrounding tissues. The gels may be used as vehicles for antibiotics, permitting protracted release of drug in the immediate environment of the implant. Antibiotic-loaded gels like this have been used in infections of the middle ear and other sites not readily reached by other methods of administration. Surgical suture material coated with antibiotic-containing hydrophilic gels acquires a chemotherapeutic role as the development of spread of infection along the suture fibre is prevented.
Structure I Poly(HEMA) crosslinked with ethylene glycol dimethacrylate (EGDMA)
Structure II Poly(HEMA) crosslinked with N, N′-methylene-bisacrylamide (BIS)
Hydrophilic contact lenses (such as Soflens) are made from crosslinked poly(2-hydroxyethyl methacrylates). The emphasis in their development has been on their permeability to oxygen. They have also been used as reservoirs for drug delivery to the corneal surface. Conventional eye drop medication (see Chapter 9) has been modified over the years through the addition to formulations of a variety of viscosity-enhancing agents, polymers such as hydroxypropylmethylcellulose (HPMC), poly(vinyl alcohol) and silicones. These all prolong contact of drug with the cornea by increasing the viscosity of the medium and retarding the drainage of the tear fluid from the eye via the punctae.
Heterogels
As it is possible to produce macromolecular chains with segments that have different solubilities in a given solvent (copolymers), one would expect that concentrated solutions of such copolymers would behave in a manner different from that of a simple polymer. In block copolymers of the type AAABBBAAA, in which A is water-soluble and B is water-insoluble, the insoluble parts will tend to aggregate. If, for instance, a polystyrene–poly(oxyethylene) copolymer, comprising 41% polystyrene and 59% poly(oxyethylene), is dissolved at 80°C in butyl phthalate (a good solvent for polystyrene), a gel with a microscopic layer structure is formed at room temperature; in nitromethane the form is somewhat different (Fig. 7.8a) as the nitromethane preferentially dissolves the poly(oxyethylene) chains.
Figure 7.8 Structure of a copolymer of type A–B made from polystyrene and polyoxyethylene (a) in nitromethane (cylindrical structure) and (b) in butyl phthalate (layer structure). Nitromethane dissolves the poly(oxyethylene) part preferentially, but butyl phthalate dissolves the polystyrene part. (–––) Polystyrene; (– – –), poly(oxyethylene); (○) solvent.
Reproduced with permission from Sadron F. Angew Chem 1963;2:248.
Poly(oxyethylene)–poly(oxypropylene)–poly(oxyethylene) block copolymers, known commercially as Pluronic or poloxamer surfactants, are used as emulsifiers. Some form micellar aggregates in aqueous solutions above a critical micelle concentration, in which the hydrophobic central block associates with other like blocks, leaving the hydrophilic poly(oxyethylene) chains to the outside and protecting the inner core. Packing of these micelles in solution of high concentration leads to the reversible formation of gels, as shown in Fig. 7.9.
Figure 7.9 ABA-type copolymers: (a) micelles in dilute solution; (b) formation of a cubic-phase gel, by packing of micelles.
Syneresis
Syneresis is the term used for the separation of liquid from a swollen gel. Syneresis is thus a form of instability in aqueous and non-aqueous gels. Separation of a solvent phase is thought to occur because of the elastic contraction of the polymeric molecules; in the swelling process during gel formation, the macromolecules involved become stretched and the elastic forces increase as swelling proceeds. At equilibrium, the restoring force of the macromolecules is balanced by the swelling forces, determined by the osmotic pressure. If the osmotic pressure decreases, as on cooling, water may be squeezed out of the gel.
|
|
7.3.3 Polymer complexes
The varied structure and chemistry of polymers provide ample opportunity for complexes to form in solution. One example occurs when an aqueous solution of high-molecular-weight polyacids is mixed with polyglycols. The viscosity and pH of the solution of the equimolar mixture of polyacid and glycol remain the same with the increase in oligomer chain length up to a critical point. The nature of the interaction is shown in (III); this occurs only when the polyethylene glycol molecules have reached a certain size.
Structure III Polycomplex formed by interaction of polyacid and polyoxyethylene glycol
Such macromolecular reactions are highly selective and strongly dependent on molecular size and conformation. On mixing, some of the macromolecules might be involved in the complex while the rest will be free. The reason for compositional heterogeneity of the products could be the conformational transitions of macromolecules in the course of complex formation.
Interactions between macromolecules can occur in formulations, for example, when preparations are mixed. They can be put to good advantage in the synthesis of novel compounds. Polyethyleneimine and poly(acrylic acid) form a polyelectrolyte complex with salt-like bonds, as shown in (IV). If the complex is heated as a film, interchain amide bonds are formed between the groups that formed electrostatic links. The non-ionised ‒COOH and ‒NH groups in the chain are the points of structural defects in the film.
Structure IV Complex formation between polyethyleneimine and poly(acrylic acid)
7.3.4 Binding of ions to macromolecules
Calcium is coordinated between certain uronic acid-containing polysaccharides (V), which can explain the tight binding of calcium and other multivalent ions in polysaccharide structures, and also how bivalent ions can induce gel formation in acidic polysaccharides such as alginic acid solutions.
Structure V Calcium complexed in polysaccharides
It has been found that such interactions have dietary significance.
|
Dietary fibre from plants binds calcium in proportion to its uronic acid content. This binding by the non-cellulosic fraction of fibre reduces the availability of calcium for small-intestinal absorption, although colonic digestion of uronic acids liberates the calcium.4 The pH dependence of the binding strongly suggests the involvement of carboxylic acid groups. Where daily fibre intakes vary between 50 and 150 g, with perhaps 30–110 mmol uronic acid, the binding capacity of fibre may exceed the total intake of calcium, which may be less than 20 mmol (800 mg) per day. |
7.3.5 Interaction of polymers with solvents including water
As a consequence of their size, polymers interact with solvents in a more complex fashion than do smaller crystalline solutes. A given polymer may have no saturation solubility; it usually either dissolves completely or is only swollen by a given liquid. If the polymer is crosslinked, solution cannot occur and the polymer will only swell by imbibition of liquid to form a gel. Swelling decreases as the degree of crosslinking increases. Swelling is also a function of the solubility parameter of the liquid phase, and if the polymer is ionic, swelling will be dependent on the ionic strength of the solution, as shown in Fig. 7.10 for crosslinked hyaluronic acid (HA) gels. Increasing ionic strength decreases the repulsion between the chains and allows the polymer to shrink.
Figure 7.10 Relative swelling of hyaluronic acid hydrogels in different concentrations of NaCl and Na2SO3 solutions.
Reproduced from Tomer R et al. Electrically controlled release of macromolecules from cross-linked hyaluronic acid hydrogels. J Control Release 1995;33:405. Copyright Elsevier 1995.
Highly polar polymers like poly(vinyl chloride) and some cellulose derivatives require polar liquids as solvents, in which dipole interactions or hydrogen bonding between polymer and solvent molecules occur. However, solvation does not necessarily lead to solution because the liquid, if it is to act as a solvent, must dissolve the solvated polymer. This process may be very slow because of the high viscosity of the partially solvated system.
Swelling of hydrogels and drug release
The relative mobility of a drug diffusing in the swelling hydrogel is given by the swelling interface number, Sw defined as
where ν is the velocity of the moving front, δ(t) is the thickness of the rubbery layer (the infiltration and gel layers represented in Fig. 7.7) at time t and D is the diffusion coefficient of the drug in the matrix.5 When Sw 1, Fickian drug diffusion predominates, whereas when Sw
1, zero-order release kinetics are observed. The amount of drug released from a thin slab is expressed as an exponential:
n varying from − 0.5 to >1.0 where Mt /M∞ is the fraction of drug and k is a constant.
It has been seen in Chapter 6 that the use of macromolecules as dispersion stabilisers depends in part on the osmotic forces arising from the interaction of solvated polymer chains as neighbouring particles approach (see Fig. 6.7). It is thus important to know how factors such as temperature and additives affect this interaction. Flory has given the free energy of dilution (the opposite process to the concentration effect discussed in section 6.2) as
where is the volume fraction of polymer, and k1 and ψ1 are heat and entropy parameters, respectively.
It is sometimes convenient to define the temperature at which a polymer of infinite molecular weight just becomes insoluble in a given solvent; this temperature is the Flory temperature or theta temperature, θ, which may also be defined by
so that substituting in equation (7.13) we obtain the relationship between ΔG1 and temperature:
ΔG1 is therefore zero at the theta temperature when deviations from ideality vanish, that is, there are no polymer–polymer or polymer–solvent interactions. When T = θ there can thus be no stabilisation as molecules will interpenetrate without net interaction and will exert no forces on each other.
Not only do most linear polysaccharides tend to form spirals in solution, but in their tendency to associate they may form double helices, as does carrageenan, for instance. Under certain conditions of concentration and temperature the double helices may associate, forming gels. Possibilities exist for complex gel formation as with carrageenan, or of xanthan gum with locust bean gum. The locust bean gum molecule can associate over part of its length with the helix of xanthan, for example, while the other part of the molecule associates with another xanthan molecule, thereby acting as a bridging agent.
The firmness or strength of gels produced by such interactions will depend on the degree of interaction of the complex with water and the properties of the bridging units.
The ability to change the swelling characteristics of a polymer gel by heat, pH or application of electric current can be valuable in specialised delivery systems, as exemplified in Fig. 7.11, which shows the effect of temperature change on the swelling and de-swelling of a hydrogel, resulting in an on–off ‘switching’ mechanism.
Figure 7.11 On–off switching mechanism for drug release.
Reproduced from Yoshida R et al. Pulsatile drug delivery systems using hydrogels. Adv Drug Deliv Rev 1993;11:85. Copyright Elsevier 1993.
The relationship between swelling and release in an electrically responsive hydrogel is shown in Fig. 7.12. The mechanism of the current-induced change in volume of the gel is shown in Fig. 7.12b.
Figure 7.12 (a) Responsive swelling (●) and release (■) from poly(styrene sulfonic acid)-loaded hyaluronic acid hydrogels when an electric field of 10 V cm−1 was switched off. (b) The effect of an electric field on a polyelectrolyte network. The redistribution of ions causes shrinkage of the gel at the cathode and expansion at the anode.
(a) Reproduced from Tomer R et al. Electrically controlled release of macromolecules from cross-linked hyaluronic acid hydrogels. J Control Release, 1995; 33: 405. Copyright Elsevier 1995.
Hydrophilic polymers as bulk laxatives
The ability of carbohydrates and other macromolecules to imbibe large quantities of water is put to use both medicinally and industrially; for example, in absorbent paper and sanitary towels, incontinence pads and surgical dressings. Medically, use is made of the swelling properties in the treatment of constipation and in appetite suppression. Three properties are of importance in the in vitro evaluation of bulk laxatives:
- the volume of water absorbed in the various media
- the viscosity and texture of the gel formed
- the ability of the gel to retain water.
The swelling properties of a sterculia-based preparation (Normacol) in various aqueous media and a comparison of Normacol with two other agents are shown in Fig. 7.13.
Figure 7.13 (a) The volume attained by 5 g of Normacol Special in various solutions over 30 hours. (b) The volumes attained by 5 g of Normacol Special, Isogel and Celevac in distilled water.
Reproduced from Ireson JD, Leslie GB. Pharm J 1970;205:540.
|
It is desirable that colloidal bulk laxatives swell in the lower part of the small intestine and in the large intestine to cause reflex peristalsis, rather than in the stomach or duodenum; that is, they should swell in neutral rather than acidic or alkaline conditions. In artificial intestinal juice, psyllium seed gum increased in volume 5–14 times, locust bean gum 5–10 times and methylcellulose 16–30 times in 24 hours. In vivo evaluation of methylcellulose and carboxymethylcellulose suggests that they have two advantages over the natural gums. Methylcellulose is more efficient as a bulk laxative because of its greater water-retentive capacity, whereas carboxymethylcellulose gives uniform distribution through the intestinal contents. |
7.3.6 Adsorption of macromolecules
The ability of some macromolecules to adsorb at interfaces is made use of in suspension and emulsion stabilisation (see Chapter 6). Gelatin, acacia, poly(vinyl alcohol) and proteins adsorb at interfaces. Sometimes such adsorption is unwanted, as in the case of insulin adsorption on to glass infusion bottles and poly(vinyl chloride) infusion containers and tubing used in giving sets.
|
Adsorption of insulin to glass bottles and plastic intravenous (IV) tubing at slow rates of infusion is well documented. Adsorption ranges from 5% to 3.1% when 20 and 40 units respectively are added to 500 cm3 of isotonic sodium chloride solution. Plastic IV tubing adsorbed 30% of 20 units and 26% of 40 units added to the same infusion bottles (Fig. 7.14). Adsorption occurs rapidly, within 15 seconds. Addition of albumin to prevent adsorption is now common practice. The albumin adsorbs at the glass or plastic surface and presents a more polar surface to the solution, thus reducing, but not always preventing, adsorption of the insulin (Fig. 7.15). The binding is considered to be a non-specific phenomenon that may occur on other inert materials such as polyethylene and glass. |
Figure 7.14 Amounts of insulin lost by adsorption to glass bottles and plastic intravenous tubing, following injection of 30 units of insulin. The patient receives only 6.36 units.
Reproduced from Petty C, Cunningham NL. Anaesthesiology 1974;40:400.
Figure 7.15 Prevention of insulin loss via adsorption by the addition of human serum albumin (HSA) or Plasmanate to 1000 cm3 of Ringer’s lactate (RL) solution in a glass bottle. Insulin (30 units) was injected and measured at 5 minutes. Values represent means ± SEM. All HSA and Plasmanate values were significantly different from Ringer’s lactate solution control (p < 0.001).
Reproduced from Petty C, Cunningham NL. Anaesthesiology 1974;40:400.
The adsorption of macromolecules at interfaces may be the reason why molecules such as those of HA can act as biological lubricants in joint fluids (see section 7.4.11). In healthy joints only 0.5 cm3 of synovial fluid is required to provide almost perfect lubrication; in diseased joints there are sometimes faults in this lubrication system and some research has been aimed at producing synthetic substitutes for synovial fluid. Polymer solutions provide one approach as their rheological characteristics more closely approach those of the natural fluid, which is non-Newtonian.
7.4 Water-soluble polymers used in pharmacy and medicine
In this section the properties of some specific polymers used in pharmacy and medicine will be discussed. This cannot be an exhaustive treatment of the subject, so choice of the macromolecular material for this section has been based partly on the degree of use but partly on the generally interesting features they display. The choice of a macromolecular material for a particular pharmaceutical use is often difficult because of the diversity of properties exhibited by the materials available. Figure 7.16 illustrates how the field can be narrowed to some extent by grouping the natural and synthetic materials of interest to the formulator. This is, however, a very general guide, as the properties of individual macromolecules will, as discussed, often vary with pH, temperature, molecular weight and ionic strength. The most readily altered variable is, of course, the concentration of the macromolecule, whose effect on viscosity is illustrated for a range of compounds in Fig. 7.17. The most viscous material shown here is Carbopol 934.
Figure 7.16 Effect of shear rate on the viscosity of gum solutions grouped according to their rheological behaviour.
Modified from Szezesniak AS, Farkas EH. Objective characterization of the mouthfeel of gum solutions. J Food Sci 1962;27:381. Copyright Wiley-VCH Verlag GmbH & Co. KGaA. Reproduced with permission.
Figure 7.17 Viscosity of solutions of some pharmaceutical polymers and gums compared with glycerol plotted as a function of concentration.
Reproduced from Martin AN et al. Rheology. Adv Pharm Sci 1964;1:1.
7.4.1 Carboxypolymethylene (Carbomer, Carbopol)
Carboxypolymethylene is used as a suspending agent in pharmaceutical preparations and as a binding agent in tablets, and it is used in the formulation of prolonged-action tablets. It is a high-molecular-weight polymer of acrylic acid, containing a high proportion of carboxyl groups. Its aqueous solutions are acidic; when neutralised the solutions become very viscous, with a maximum viscosity occuring between pH 6 and 11. Electrolytes reduce the viscosity of the system and thus high concentrations of the polymer have to be employed in vehicles where ionisable drugs are present. A carbomer gel prolongs the contact time of drugs instilled into the eye.
7.4.2 Cellulose derivatives
Cellulose itself is virtually insoluble in water, but aqueous solubility can be conferred by partial methylation or carboxymethylation of cellulose.
Ethylcellulose is an ethyl ether of cellulose containing 44–51% of ethoxyl groups. It is insoluble in water but soluble in chloroform and in alcohol. It is possible to form water-soluble grades with a lower degree of substitution.
Methylcellulose samples are prepared by heterogeneous reaction that is usually controlled to allow substitution of, on average, about one-half of the hydroxyl groups. This leads to a product in which the methylated groups are not evenly distributed throughout the chains; rather, there are regions of high density of substitution (as in structure VI) that are hydrophobic in nature, and regions of low density of substitution that are hydrophilic in nature.
Structure VI Highly methylated region of the methylcellulose chain
Methylcellulose is thus a methyl ether of cellulose containing about 29% of methoxyl groups; it is slowly soluble in water. A 2% solution of methylcellulose 4500 has a gel point of about 50°C. High concentrations of electrolytes salt out the macromolecules and increase their viscosity; eventually precipitation may occur. Low-viscosity grades are used as emulsifiers for liquid paraffin and other mineral oils. High-viscosity grades are used as thickening agents for medicated jellies and as dispersing and thickening agents in suspensions.
Since methylcelluloses are poorly soluble in cold water, preliminary use of hot water ensures wetting of all portions of the particle prior to solution in cold water. The water-soluble methylcelluloses possess the property of thermal gelation; that is, they gel on heating while the natural gums gel on cooling. Methylcellulose exists in solution as long thread-like molecules hydrated by water molecules. On heating, the water of solvation tends to be lost; the ‘lubricating’ action of the hydration layer is also lost and the molecules lock together in a gel. Gelation is reversible on cooling. Variation in the alkyl or hydroxyalkyl substitution can be a means of controlling the gel points (Table 7.4). As the methoxyl content is lowered, the temperature of gelation increases and water solubility decreases. Unlike the ionic celluloses, the non-ionic alkylcelluloses possess surface activity. As the methoxyl content is reduced, the surface and interfacial activities are also reduced, reflecting the importance of the hydrophobic moiety in determining surface activity.
Table 7.4 Gel point and surface activity of cellulose derivatives
Derivative | Percentage ‒OCH3 | Percentage ‒OCH2CH(OH)CH3 | Gel pointa (°C) | Surface tensionb (mN m−1) | Interfacial tensionc (mN m−1) |
Methocel MC | 27.5–3.2 | – | 50–55 | 47–53 | 19–23 |
Methocel 60HG | 28–30 | 7–12 | 55–60 | 44–50 | 18–19 |
Methocel 65HG | 27–30 | 4–7.5 | 60–65 | – | – |
Methocel 70HG | 24–27 | 4–8 | 66–72 | – | – |
Methocel 90HG | 22–25 | 6–12 | 85 | 50–56 | 26–28 |
Reproduced from Windover FE. In: Davidson RL, Sittig M (eds) Water Soluble Resins. New York: Reinhold; 1962: 52ff.
a2% solution.
bSurface tension at 25°C.
cInterfacial tension versus paraffin oil at 25°C.
Ethylhydroxyethylcellulose (EHMC) is an ether of cellulose with both ethyl and hydroxyethyl substituents attached via ether linkages to the anhydroglucose rings. It swells in water to form a clear, viscous colloidal solution. Preparation of solutions of cellulose derivatives requires hydration of the macromolecules, the rate of which is a function of both temperature and pH, as shown in the example in Fig. 7.18.
Figure 7.18 Effect of pH and temperature on the hydration time of fast-dissolving grades of hydroxyethylcellulose.
Reproduced with permission from Whistler RL (ed.) Industrial Gums, 2nd edn. New York: Academic Press; 1973.
Hydroxyethylcellulose (HEC) is soluble in hot and cold water but does not gel. It has been used in ophthalmic solutions. More widely used for the latter, however, is HPMC (hypromellose), which is a mixed ether of cellulose containing 27–30% of ‒OCH3 groups and 4–7.5% of ‒OC3H6OH groups. It forms a viscous colloidal solution. There are various pharmaceutical grades. For example, hypromellose 20 is a 2% solution that has a viscosity between 15 and 25 cSt (centistokes) at 20°C; the viscosity of a 2% hypromellose 15000 solution lies between 12 000 and 18 000 cSt. Hypromellose prolongs the action of medicated eye drops and is employed as an artificial tear fluid.
Sodium carboxymethylcellulose (NaCMC) is soluble in water at all temperatures. Because of the carboxylate group, its mucilages are more sensitive to change in pH than are those of methylcellulose. The viscosity of an NaCMC mucilage is decreased markedly below pH 5 or above pH 10.
7.4.3 Natural polymers: gums and mucilages
Gum arabic (acacia) has been used traditionally in pharmacy as an emulsifier. It is a polyelectrolyte whose solutions are highly viscous owing to the branched structure of the macromolecular chains; its adhesive properties are also believed to be due to, or in some way related to, this branched structure. Molecular weights between 200 000 and 250 000 Da (Mn) have been determined by osmotic pressure, values between 250 000 and 3 × 106 Da by sedimentation and diffusion, and values of 106 Da by light-scattering measurements which also point to the shape of the molecules as short stiff spirals with numerous side-chains. Arabic acid prepared from commercial gum arabic by precipitation is a moderately strong acid whose aqueous solutions have a pH of 2.2–2.7.
Whereas most gums are very viscous in aqueous solution, gum arabic is unusual in that, being extremely soluble, it can form solutions over a wide range of concentrations up to about 37% at 25°C. The marked variation in viscosity means that the gum arabic molecules must be flexible, with the ionic acid carboxyl groups distributed along the chain. At low pH the carboxyl groups are un-ionised. On increase of pH the carboxyl groups become progressively ionised and the folded chains expand owing to repulsion between the charged groups, causing an increase in viscosity. On addition of NaOH to the system the viscosity falls again as the concentration of counterion (Na+) increases and effectively shields the acidic groups. The molecule then folds on itself. Similar falls in viscosity are exhibited on addition of sodium chloride. The effect of salt addition to the gum at fixed pH reflects the decrease in effective charge on the molecules of gum with resultant contraction and reduction in viscosity.
The gum arabic molecule is, in addition, surface-active: a 4% solution at 30°C has a surface tension of 63.2 mN m−1. Addition of electrolytes makes the molecule more surface-active either by causing a change in conformation of the molecule at the interface, allowing closer packing, or by increasing the hydrophobicity of the molecule. It is an effective emulsifier, the stabilisation of the emulsion being dependent mainly on the coherence and elasticity of the interfacial film, which is by no means monomolecular.
Gum arabic is incompatible with several phenolic compounds; under suitable conditions it forms coacervates (see section 7.6.3) with gelatin and positively charged polyelectrolytes.
Gum tragacanth partially dissolves in water; the soluble portion is called tragacanthin and this can be purified by precipitation from water with acetone or alcohol. Tragacanthin is a highly viscous polyelectrolyte with a molecular weight of 800 000 Da (as determined by sedimentation measurements). It is one of the most widely used natural emulsifiers and thickeners. As its molecules have an elongated shape, its solutions have a high viscosity which, as with gum arabic, is dependent on pH. The maximum viscosity occurs at pH 8 initially, but because of ageing effects the maximum stable viscosity is near pH 5.
It is an effective suspending agent for pharmaceuticals and is used in conjunction with acacia as an emulsifier, the tragacanth imparting a high structural viscosity while the gum arabic adsorbs at the oil–water interface.
|
Tragacanth is also used in spermicidal jellies, acting both by immobilising spermatozoa and acting as a viscous barrier to spermatozoa. It is also used as an excipient in contraceptive preparations which contain chemical contraceptive molecules where its physical effect on spermatozoa reinforces the direct chemical action of the contraceptive agents. |
Alginates. Although the solutions of alginate are very viscous and set on addition of acid or calcium salts, they are less readily gelled than pectin and are used chiefly as stabilisers and thickening agents. Propylene glycol alginate does not precipitate in acid and, as it is non-toxic, is widely used as a stabiliser for foodstuffs. The molecules are highly asymmetric, with molecular weights in the range 47 000–370 000 Da. Sodium alginate has the structure given in VII.
Structure VII Sodium alginate
Pectin is a purified carbohydrate product from extracts of the rind of citrus fruits and consists of partially methoxylated polygalacturonic acid (VIII). It has remarkable gelling qualities but is also used therapeutically, often with kaolin, in the treatment of diarrhoea. It has been established that the longer the pectin chains, the greater its capacity for gel formation. The presence of inorganic cations and the degree of esterification of the carboxyl groups are important factors; in the case of calcium pectate gel it can be assumed that the calcium or indeed other polyvalent cations can interlink the chains by binding through COO−…Ca2+…−OOC interactions. Thus a high degree of esterification will disfavour gelation in this case. In the absence of inorganic cations, however, a high degree of esterification aids gelation, suggesting that hydrophobic interactions cause the chains to associate.
Structure VIII Partially methylated chain of poly(galacturonic acid) of pectin
7.4.4 Chitosan
Chitosan is a polymer obtained by the deacetylation of chitin, one of the most abundant polysaccharides. Chitosan, or poly[α-(1,4)-2-amino-2-deoxy-D-glucopyranose], has the structure (IX). As might be expected, the degree of deacetylation has a significant effect on the solubility and rheological properties of the polymer. At low pH, the polymer is soluble, with the sol–gel transition occurring at approximately pH 7. Chitosan also has film-forming abilities and its gel- and matrix-forming abilities make it useful for solid-dosage forms, such as granules or microparticles.6 The molecular weight, crystallinity and degree of deacetylation are all factors that can be varied to control the release rates from chitosan-based granules.
Structure IX The chemical structure of chitosan. The amine groups on the polymer have a pKa in the range 5.5–6.5, depending on the source of the polymer
7.4.5 Dextran
Certain fractions of partially hydrolysed dextran are used as plasma substitutes or ‘expanders’. Certain strains of Leuconostoc meserentoides are cultivated to synthesise dextran (X), which is a polymer of anhydroglucose, linked through α-1,6 glucosidic linkages. The chains are branched; on the average, one branch occurs for every 10–12 glucose residues. The intrinsic viscosity [] is related to M by the relationship
in the molecular weight range 20 000–200 000 Da. The dextrans produced by fermentation are hydrolysed and fractionated to give a range of products suitable for injection. Dextran, being a hydrophilic colloid, exerts an osmotic pressure comparable to that of plasma and it is thus used to restore or maintain blood volume. Other substances that have been used in a similar way include hydroxyethyl starch, polyvinylpyrrolidone (PVP) and gelatin.
Structure X Dextran
|
Dextran injections are sterile solutions of dextran with weight-average molecular weights of about 40 000–110 000 Da. Dextrans with a molecular weight of about 50 000 Da or less are excreted in the urine within 48 hours of injection. Dextran molecules with higher molecular weights disappear more slowly from the blood stream and are temporarily stored in the reticuloendothelial system. Dextran 70 (70 000 Da) and Dextran 110 (110 000 Da) are used to maintain blood volume, and Dextran 40 is used primarily to prevent intravascular aggregation of blood cells and for assisting capillary blood flow. This latter effect is the result of dextran adsorption and stabilisation of the erythrocyte suspensions. If the higher-molecular-weight fractions exceed about 1% concentration in the blood, rouleaux (see photomicrograph) tend to form. |
The sensitivity of blood to the concentration and molecular weight of dextran is clearly seen in Fig. 7.19, where aggregation and relative viscosity of red-cell suspensions are shown in the presence of varying amounts of five different dextrans. Molecular weight control is thus important and may be exercised by measurement of intrinsic viscosity, [].
Figure 7.19 Variation of indices of red-cell aggregation with the concentration of five dextran fractions of different molecular weight: (a) microscopic aggregation index; (b) relative viscosity at a shear rate of 0.1 s−1. Note that the maximum of each curve (that is, maximum aggregation of the red cells) corresponds to a well-defined concentration of a particular dextran fraction. Maxima in both indices of aggregation of red cells occur at about the same concentration of dextran fraction.
Reproduced with permission from Chien S. Bib Anat Basel 1973;11:244.
7.4.6 Iron–dextran complexes
Iron–dextran complexes are soluble, non-ionic and suitable for injection for the treatment of anaemia (Fig. 7.20); the complex is stable on storage in the pH range 4–11.
Figure 7.20 Conceptual drawing of an iron–dextran complex, showing the iron oxide core surrounded by a corona of dextran and carboxydextran molecules.
After Abdollah MRA et al. Faraday Disc 2014; 75:41–58; see also London E. J Pharm Sci 2004;93:1838–1846.
7.4.7 Polyvinylpyrrolidone
PVP is used as a suspending and dispersing agent, as a tablet-binding and granulating agent, and as a vehicle for drugs such as penicillin, cortisone, procaine and insulin to delay their absorption and prolong their action. It forms hard films that are utilised in film-coating processes. Chemically it is a homopolymer of N-vinylpyrrolidone (XI). It is available in a number of grades, designated by numbers ranging from K15 to K90. The K values represent a function of the mean molecular weight, and can be calculated from
where c = concentration in grams per 100 cm3 and rel is the viscosity relative to the solvent. K = 1000K0. Viscosity is essentially independent of pH over the range 0–10 and aqueous solutions exhibit a high tolerance for many inorganic salts. Its wide solubility in organic solvents is unusual. The viscosity of a range of aqueous solutions of PVP is shown in Fig. 7.21.
Structure XI Polyvinylpyrrolidone
Figure 7.21 Viscosity of polyvinylpyrrolidone (PVP) solutions as a function of molecular weight (PVP K15 (mol wt 40 000 Da) to PVP K90 (mol wt 700 000 Da)) and concentration of the polymer in water.
Reproduced from Azorlosa JL, Martinelli AJ. In: Davidson RL, Sittig M (eds) Water Soluble Resins. New York: Reinhold; 1962.
PVP forms molecular adducts with many substances. Soluble complexes, called iodophors, are formed with iodine: the solubility of iodine is increased from 0.034% in water at 25°C to 0.58% by 1% PVP. The resulting iodophor retains the germicidal properties of iodine. It is thought that the iodine is held in a PVP helix in solution. The influence of two samples of PVP on the solubility of testosterone is shown in Fig. 7.22. The PVP correspondingly increases the rate of solution of the steroid from solid dispersions.
Figure 7.22 The influence of PVP 11500 and PVP 40000 on the aqueous solubility of testosterone at 37°C.
Reproduced from Hoelgaard A, Muller N. Arch Pharm Chem 1975;3:34.
7.4.8 Polyoxyethylene glycols (macrogols)
Poloxyethyelene glycols are widely used in pharmaceutical formulations not only as solvents and bases for ointments and suppositories but also as molecules which can determine the lifetime of nanoparticles in the blood by adsorbing on to particle hydrophobic surfaces to prevent opsonin adsorption (see Chapter 14).
Macrogols (polyoxyethylene glycols (PEGs), XII) are liquid over the molecular weight range 200–700 Da; the liquid members and semisolid members of the series are hygroscopic. Macrogol 200 has a hygroscopicity 70% of that of glycerol, but this decreases with molecular weight: the comparable value for Macrogol 1540 is only 30%. They are used as solvents for drugs such as hydrocortisone. The macrogols are incompatible with phenols and can reduce the antimicrobial activity of other preservatives. Higher-molecular-weight PEGs are more effective on a molecular basis as complexing agents. Up to four phenol molecules bind to each PEG molecule; the complex formed is of the donor–acceptor type. The semisolid and waxy members of the series may be used as suppository bases; in such cases their potential to interact with medicaments must be borne in mind.
Structure XII Polyoxyethylene glycol
Use of PEGs, and other hydrophilic polymers, in high concentrations in formulations can influence the behaviour of drugs even when the drug is present as a physical mixture with the polymer. For example, combination of PEG 4000 with sulfathiazole increases the solution rate of sulfonamides (Fig. 7.23). Probable mechanisms include an increase in drug solubility or increased wetting of the drug surrounded by the hydrophilic polymer. Apart from these uses, the PEGs have turned out to be one of the most versatile water-soluble polymers. They have been successful in modifying the behaviour of proteins, including monoclonal antibodies, in solution and in the circulation after being grafted to these molecules. The PEGs also prolong the circulation time of particulate delivery systems such as liposomes and nanoparticles through their adsorption or incorporation into the surface of these carriers, preventing the adsorption of opsonins and reducing uptake by the liver and spleen, as discussed in Chapter 12.
Figure 7.23 Dissolution rates of sulfathiazole (Form I)–polyethylene glycol 4000 physical mixtures.
Reproduced from Niazi S. Effect of polyethylene glycol 4000 on dissolution properties of sulfathiazole polymorphs. J Pharm Sci 1976;65:302. Copyright Wiley-VCH Verlag GmbH & Co. KGaA. Reproduced with permission.
7.4.9 Bioadhesivity of water-soluble polymers
Adhesion between a surface of a hydrophilic polymer, or a surface to which a hydrophilic polymer has been grafted or adsorbed, and a biological surface arises from interactions between the polymer chains and the macromolecules on the mucosal surface. From Fig. 7.24a it is clear that to achieve maximum adhesion there should be maximum interaction between the polymer chains of the bioadhesive (A) and the mucus (B). The charge on the molecules will be important, and for two anionic polymers maximum interaction will occur when they are not charged. Penetration and association must be balanced. Table 7.5 shows the adhesive performance of a range of polymers, many of which have been discussed in this chapter. Of these, two classes have been approved by the US Food and Drug Administration: anionic poly(acrylic acid) (carbophil) derivatives and the cationic chitosans. Polycarbophil and carbomer (Carbopol 934P) have pKa values of about 4.5 and display maximum mucoadhesivity at pH values where they are mostly undissociated7 (Fig. 7.24b).
Figure 7.24 (a) Schematic representation of two phases, adhesive (A) and mucus (B), which adhere owing to chain adsorption and consecutive chain entanglement during mucoadhesion. (b) Effect of pH on in vitro bioadhesion of polycarbophil to rabbit gastric tissue.
(a) Reproduced from Peppas NA, Mikos AG. In: Gurney R, Junginger HE (eds) Bioadhesion. Stuttgart: Wiss. Verlagsgesellschaft; 1990. Copyright Elsevier 1990.
(b) From reference 7.
Table 7.5 Some representative mucoadhesives and their relative mucoadhesivities
Substance | Adhesive performance |
Carboxymethylcellulose | Excellent |
Carbopol | Excellent |
Carbopol and hydroxypropylmethylcellulose | Good |
Carbopol base with white petrolatum/hydrophilic petrolatum | Fair |
Carbopol 934 and EX 55 | Good |
Poly(methyl methacrylate) | Excellent |
Polyacrylamide | Good |
Poly(acrylic acid) | Excellent |
Polycarbophil | Excellent |
Homopolymers and copolymers of acrylic acid and butyl acrylate | Good |
Gelatin | Fair |
Sodium alginate | Excellent |
Dextran | Good |
Pectin | Poor |
Acacia | Poor |
Povidone | Poor |
Poly(acrylic acid) crosslinked with sucrose | Fair |
7.4.10 Viscous solutions and disorders of the eye
Anterior-chamber injections (intracameral injections)
Hypotony is defined as an intraocular pressure of 5 mmHg or less. Low intraocular pressure can adversely impact the eye in many ways, including causing accelerated cataract formation, maculopathy and discomfort.
Sodium hyaluronate, chondroitin sulfate and methylcellulose have been compared for maintaining the form of the anterior chamber of the eye in cases of hypotony. The rheological characteristics of the polymers used in the anterior chamber are key to success. Pseudoplastic fluids are ideal for maintaining the chamber form since they are more viscous at rest. Sodium hyaluronate and methylcellulose are pseudoplastic, while chondroitin sulfate displays Newtonian flow properties.
Figure 7.25 A diagram of the forces on an intraocular lens (IOL). The applied force on the lens is represented by two vectors: the compression force and the shear force. High viscosity transmits more of the shear force to the endothelial surface. The compression force is minimised by a high viscosity (note the similarity to synovial fluid) and the drag force is minimised by low viscosity.
High viscosity is critical when the agent is applied in a thick layer to prevent mechanical damage to the corneal epithelium when an intraocular lens is drawn across the endothelium. Compression and shear are responsible for the damage8: thin layers of highly viscous HA convey the shear forces to the endothelium, whereas thick layers provide a physical barrier to compression, as can be seen in Fig. 7.25. Clearly there are analogies to synovial fluid here.
Optiflex is one ophthalmic product containing sodium hyaluronate with a molecular weight of 4 × 106 Da in a sterile isotonic vehicle. When injected through a cannula it becomes less viscous, but it regains its viscosity in the anterior chamber of the eye. It is used for lubrication and protection of cells and tissue during surgical procedures.
Dry eye
In some patients, the supply of tear fluid (as discussed in Chapter 9), which comprises a largely aqueous solution containing protein, lipids and enzymes, is impaired. Tears spread over the surface of the cornea, which is hydrophobic. Tear films thin and break but the fluid is replenished by blinking. The film break-up time is variable but is more rapid in patients suffering from dry-eye syndrome. The tears contain phospholipids, which act as a surfactant, lowering the surface tension of the fluid and allowing spreading. The thin lipid layer on the surface of the tear film (like most lipid monolayers) also slows down evaporation of the aqueous medium beneath. Dry eye9 (xerophthalmia*; Sjögren’s syndrome) is caused when the tear film thins to such an extent that it ruptures, exposing the corneal surface to the air, as shown schematically in Figure 7.26. Drying out of patches on the corneal surface follows. This can be painful and must be avoided. Evaporation over 5–10 minutes can actually eliminate the tear film completely.10
Figure 7.26 Schematic of (a) the effect of the lipid layer reducing evaporation of tear fluid; (b) the beginning of the thinning process; and (c) the eventual separation of the tear liquid, inducing the formation of dry spots on the cornea.
Artificial tear fluids may replace the natural tear fluid with varying degrees of similitude. Most aim for formulations that have an appropriate viscosity, but these do not necessarily possess identical rheological properties to those of natural tear fluids. HPMC is a component of many commercial replacement or ‘artificial’ tear products, as are the water-soluble macromolecules NaCMC (carmellose), poly(vinyl alcohol) and PVP.
Xerostomia
Another syndrome that requires replacement or supplementation of a natural fluid with an artificial substance is xerostomia (‘dry mouth’). Normal saliva function and control are compromised in this condition. Saliva is a clear, usually alkaline and somewhat viscous secretion from the parotid, submaxillary, sublingual and smaller mucous glands of the mouth. In some cases xerostomia is caused by medication, especially by anticholinergics. Chemotherapeutic agents can also have a direct effect on salivary glands, reducing saliva output. Saliva consists primarily of water but contains enzymes and other proteins and electrolytes. It has a surface tension of around 58 mN m–1. Saliva is essential for the normal ‘feel’ of the mouth and it assists lubrication, possesses antimicrobial activity and aids mucosal integrity. Saliva provides protection by constantly flushing non-adhered microbes, their toxins and nutrients from the mouth. It has also been suggested that the flow of saliva detaches adsorbed microbes from the teeth or prevents their adhesion, as shown in Fig. 7.27 Saliva contains a wide spectrum of agents such as lactoferrin, lysozyme, histatins, cystatins, mucins, agglutinins, secretory leukocyte proteinase inhibitor, tissue inhibitors of proteinases, chitinase, peroxidases and calprotectin.
Figure 7.27 Diagram of the effect of salivary flow in the normal mouth, dislodging bacteria adsorbed on teeth. Different situations are shown, with more exposed bacteria and bacteria in sheltered sites, such as crevices.
Dry mouth can be treated with artificial saliva, although these solutions, as can be imagined from the list of components of natural saliva, seldom truly mimic the properties of the natural lubricant and wetting material. Designed to behave as far as possible like natural saliva, commercially available artificial salivas mostly contain carboxymethylcellulose and hydroxyethylcellulose to increase viscosity. The rheological properties of saliva are, as one can imagine, quite complex, but these polymer additives do at least increase the residence time of the fluid. However, gel formulations that can prolong contact between the fluid and the oral mucosa are sometimes preferred.
7.4.11 Intra-articular hyaluronic acid
Synovial fluid is rheopexic – that is, stress increases with time in steady shear (see section 6.3). This is thought to be the result of protein aggregation with time and the influence of stress on the process. It is suggested11 that there is a connection between the observed rheopexy and the remarkable lubrication properties of synovial fluid; one can envisage that the fluid that exists between two bony surfaces becomes more effective in ‘cushioning’ the contacts as its viscosity increases with the forces placed on it.
Products containing HA, such as Hyalgan, Artzal, Synvisc, Suplasyn, Hyalart and Orthovisc, have been developed for administration into the synovial space of joints to enhance the activity of the natural synovial fluid. The perception that higher-molecular-weight HA is superior to lower-molecular-weight species is based on the suggestion that high-molecular-weight HA normalises synovial fluid and results in effective joint lubrication. Higher-molecular-weight HAs are chemically crosslinked forms and are claimed to have a greater residence time in the joints.12 In vivo, HA with a higher viscosity has been found to be more effective in lubricating joints.13
There is some controversy about modes of action. It has also been suggested that viscoelasticity does not in fact form the foundation of the beneficial properties of these injections.14 This point is added because explanations of the behaviour of complex systems are fraught with confounding factors. One must, however, speculate from a reasoned base when necessary. It is highly reasonable to conclude that one of the important properties of hyaluronan and hyaluronan solutions is their pseudoplastic behaviour. These then serve as lubricants when joint movements are slow and as shock absorbers when movements are fast15 (Fig. 7.28).
Figure 7.28 The site of administration of intra-articular injections of agents such as hyaluronic acid to lubricate the movement of the joints, as discussed in the text.
7.4.12 Polymer crystallinity
Polymers form perfect crystals with difficulty, simply because of the low probability of arranging the chains in a regular fashion, especially at high molecular weights. Advantage can be taken of defects in crystals in the preparation of microcrystals. Microcrystalline cellulose (Avicel) is prepared by disruption of larger crystals. It is used as a tablet excipient and as a binder-disintegrant. Dispersed in water, it forms colloidal gels, and it can be used to form heat-stable oil-in-water (o/w) emulsions. Spheroids of microcrystalline cellulose with accurately controlled diameters can be prepared and drugs can be incorporated during preparation. The concept of crystallinity is potentially important when considering polymer membranes, as discussed below.
7.5 Water-insoluble polymers and polymer membranes
In defining the properties of polymers for drug formulation, certain characteristics are important. Obviously molecular weight and molecular weight distribution must be known, as these affect solvent penetration and crystallinity. The functionality of the polymer is best described by a series of parameters such as
- glass transition temperature, Tg
- tensile strength
- diffusion coefficient
- hardness (crystallinity)
- solubility.
Crystallinity defines several features of polymers: rigidity, fluidity, the resistance to diffusion of small molecules in the polymer, and degradation.
In hydrogels Tg can be measured and is a measure of polymer structure, crosslinking density, solvent content and polymer–solvent interactions, as can be seen from Table 7.6.
Table 7.6 Effect of hydrogel structure and characteristics on Tg
Feature | Effect on Tg |
Presence of flexible groups in main chain | ↓ |
Bulky, inflexible side-chains | ↑ |
Flexible side-chains | ↓ |
Increase in main-chain polarity | ↑ |
Increase in crosslinking | ↑ |
Plasticiser content | ↓ |
7.5.1 Permeability of polymers
Hydrophobic polymers also play an important role in pharmacy. When these materials are used as membranes, containers or tubing material, their surfaces may come into contact with solutions. The surfaces of insoluble polymers are not as inert as might be thought. The interaction of drugs and preservatives with plastics depends on the structure of the polymer and on the affinity of the compound for the plastic. The latter is determined by the degree of crystallinity of the polymer, as permeability is a function of the degree of amorphous content of the polymer. The crystalline regions of the solid polymer present an impenetrable barrier to the movement of most molecules. Diffusing molecules thus have to circumnavigate the crystalline islands, which act as obstructions. The greater the volume fraction of crystalline material (), the slower the movement of molecules.
Diffusion
Diffusion in a non-porous solid polymer is of course a more difficult process than in a fluid because of the necessity for the movement of polymer chains to allow passage of the drug molecule, and it is therefore slower. The equation that governs the process is Fick’s first law (see section 2.6, equation 2.63):
where J is the flux, D is the diffusion coefficient of the drug in the membrane and dc/dx is the concentration gradient across the membrane. If the membrane is of thickness l, and Δc represents the difference in solution concentration of drug at the two faces of membrane,
where K is the distribution coefficient of the permeant towards the polymer. Therefore, alteration of polymer/membrane thickness, coupled with appropriate choice of polymer, can give rise to the desired flux. Within a given polymer, permeability is a function of the degree of crystallinity, itself a function of polymer molecular weight. If P is the permeability of drug in a partially crystalline polymer (see Fig. 1.18, Chapter 1), the volume fraction of the crystalline regions being , and Pa is the permeability in an amorphous sample, then
Permeation of drug molecules through the solid polymer, which may be acting as a drug depot, is a function of the solubility of the drug in the polymer as
Addition of inorganic fillers in which the drug is insoluble alters the overall solubility of the drug in the polymer and hence alters the permeation characteristics. The equation for overall solubility (S) is given by
where f refers to filler and p to polymer. Thus, when Sf → 0, as happens when inorganic fillers such as zinc oxide are employed, an obstruction-type equation may be written:
The natural permeabilities of polymers vary over a wide range and this widens the choice, provided one can select a polymer that is compatible with the tissues with which it comes into contact. Once a polymer has been chosen that gives a flux of drug sufficient to provide adequate circulating levels, use of fillers and plasticisers can give fine control of permeability (Table 7.7).
Table 7.7 Factors that influence diffusivity in polymers
Factor | Net effect on D |
Increased polymer molecular weight | ↓ |
Increased degree of crosslinking | ↓ |
Diluents and plasticisers | ↑ |
Fillers | ↓ |
Increased crystallinity of polymers | ↓ |
Increased drug molecular size | ↓ |
The method of preparation also influences the properties of the film. Cast films of varying properties can be prepared by variation inter alia of the solvent power of the casting solution containing the polymer, although the complex processes involved in film formation are not yet fully understood. It is clear, however, that the conformation of the polymer chains in concentrated solution just prior to solvent evaporation will determine the density of the film, and the number and size of pores and voids. Drug flux through dense (non-porous) polymer membranes is by diffusion; flux through porous membranes will be by diffusion and by transport in solvent through pores in the film. With porous films, control can be exercised on porosity, and hence overall permeability, by the use of swelling agents. Dense membranes can be subjected to certain postformation treatments such as thermal annealing that modify their structural and performance characteristics.
The solubility of sterilising gases in polymers is important in determining the retention of residues that may, as in the case of ethylene oxide residues, be toxic. The quality control problems of polymers and plastics are considerable. Both the chemical and physical natures of the material have to be taken into account, as well as purity.
Permeability of polymers to gases
The permeability of polymers to the gaseous phase is of importance when the use of polymers as packaging materials is considered. Figure 7.29 shows oxygen penetration through a wide range of plastic materials, ranging from Teflon to dimethylsilicone rubber, which has the highest permeability. Ether, nitrous oxide, halothane and cyclopropane diffuse through silicone rubbers, and general anaesthesia in dogs has been achieved by passing the vapours of these substances through a coil of silicone rubber tubing, each end of which is placed in an artery or vein. Aspects of the permeability of polymeric films of interest pharmaceutically include the process of gas diffusion, water sorption and permeation and dialysis processes. With few exceptions, there is an inverse relationship between water-vapour transmission and oxygen permeability. Water-vapour permeability has been shown to be dependent on the polarity of the polymer. More polar films tend to be more ordered and less porous, hence less oxygen-permeable. The less polar films are more porous, permitting the permeation of oxygen but not necessarily of the larger water molecules. Being more lipophilic, the less polar films have less affinity for water. Because of the importance of water as a solvent and permeant species, much work has been directed towards the synthesis of polymeric membranes with controlled hydrophilic/hydrophobic balance. The hydrophilicity of the cellulose acetates is directly proportional to their ‒OH content and inversely proportional to the hydrophobic acetyl content (Table 7.8). Alternative approaches to alteration of characteristics of water permeability include the use of block copolymers where one can alter the ratio of hydrophilic polymer to increase transport rates of polar materials.
Figure 7.29 Permeation of oxygen through several polymer films. This illustrates the diversity of the properties of available polymers and shows the relatively high permeability of polydimethylsiloxane, a common component of prolonged-action drug devices.
Reproduced from Kesting R. Synthetic Polymeric Membranes. New York: McGraw-Hill; 1971.
Table 7.8 Effect of hydroxyl and acetyl content on water permeability and sorption of moisture by cellulose acetates
Hydroxyl content (%) | Acetyl content (%) | Moisture sorption (%) at 95% relative humidity at 25°C | Water permeability D1C1 (10−7 g cm s−1) |
7.2 | 34 | 19 | 12 |
5.9 | 36 | 17 | 7 |
4.6 | 38 | 14.7 | 4 |
3.3 | 40 | 12.6 | 2.5 |
2.0 | 42 | 10.5 | 1.5 |
Reproduced from Reid C, Breton E. Water and ion flow across cellulosic membranes. J Appl Polymer Sci 1959;1:133. Copyright Wiley-VCH Verlag GmbH & Co. KGaA. Reproduced with permission.
The affinity of drugs for plastics
Drugs may adsorb on to the surface of plastics or they may diffuse into the interior of the material. The affinity of drugs for plastics will vary with the structure of the drug: proteins, polypeptides and other hydrophilic or amphipathic molecules may adsorb, and smaller hydrophobic molecules sorb, that is enter the plastic. Chlorpromazine, for example, has a very high affinity for some materials used as tubing (Table 7.9). Silicone is actually very permeable to hydrophobic molecules and this can be put to good use in other areas, such as in controlled-release systems. The downside is that some drugs, e.g. steroids, are adsorbed from solutions passing through polyethylene tubing. In analytical chemistry such interactions can also be important in defining the accuracy of the method. Glyceryl trinitrate, which has a high affinity for lipophilic plastics, migrates from tablets in contact with plastic liners in packages, causing a reduction of the active content of many tablets to zero. This peculiar migratory behaviour is due to the volatility of the drug; normally the drug molecules would only be able to be significantly affected by such transfer when in the solution state.
Table 7.9 Concentration of chlorpromazine in buffer solution after shaking with various polymers for 1 h at 22°C (original concentration 100 μmol dm−3)
Material | Chlorpromazine concentration (μmol dm−3) |
Silicone tubing | 1 ± 0.4 |
Latex tubing | 16 ± 4.7 |
Poly(vinyl chloride) tubing | 14 ± 1.0 |
Polyethylene tubing | 60 ± 2.0 |
Polyethylene test tube | 70 ± 3.8 |
Polyethylene stopper | 77 ± 5.3 |
Plexiglas chippings | 74 ± 4.3 |
Teflon chippings | 81 ± 4.4 |
Polystyrene test tube | 89 ± 1.2 |
Reproduced with permission from Krieglstein G et al. On the interaction of various drugs with synthetic materials used in pharmacological apparatus. Arzneim Forsch 1972;22:1538.
7.5.2 Ion-exchange resins
Synthetic organic polymers comprising a hydrocarbon crosslinked network to which ionisable groups are attached have the ability to exchange ions attracted to their ionised groups with ions of the same charge present in solution (Fig. 7.30). These substances, usually prepared in the form of beads, are ion-exchange resins and are insoluble in water, the aqueous phase diffusing into the porous resin beads. Because ions must diffuse into and out of the resin for exchange to occur, ions larger than a given size may be excluded from reaction by altering the nature of the crosslinks in the polymer. The resins may be either cation exchangers in which the resin ionisable group is acidic, for example, sulfonic, carboxylic (XIII) or phenolic groups, or anion exchangers in which the ionisable group is basic, either amine or quaternary ammonium groups.
Figure 7.30 Schematic diagram of a cation-exchange resin framework with fixed exchange sites prior to and following an exchange reaction: (a) initial state prior to exchange reaction with cation B+; (b) equilibrium state after exchange reaction with cation B+.
Modified from Weber WJ. Physicochemical Processes for Water Quality Control. New York: Wiley; 1972. Copyright Wiley-VCH Verlag GmbH & Co. KGaA. Reproduced with permission.
Structure XIII Sulfonic acid and carboxylic acid ion-exchange resins
The equations describing the equilibria involved are as follows:
Cation-exchange resin
Anion-exchange resin
The equilibrium constant for the cation exchange resin is
However, application of equation (7.24) is impossible because of the inaccessibility of the terms [POL-B+] and [POL-A−]. Some estimation of a resin’s affinity for ions can be made using a standard ion such as lithium for cation-exchange resins. A selectivity coefficient, k, may be defined as
Even here there is a problem arising from the difficulty in the determination of the activity of the ions in the resin (because of the complexity of the environment) and the overall concentration of ion is generally used instead.
The ability of a resin to exchange one ion for another depends on its affinity for the ion and the concentration of ions in solution. Cation-exchange resins tend to have affinity in decreasing order for calcium, potassium, sodium, ammonium and hydrogen ions.
Pharmaceutical ion-exchangers
Anion-exchange resins such as polyamine methylene resin and polyaminostyrene have been used as antacids.
A polymeric ion-exchange resin, sevelamer (XIV), is used to reduce phosphate ion levels in patients with hyperphosphataemia following haemodialysis. It is a copolymer of 2-(chloromethyl)oxirane (epichlorohydrin) and prop-2-en-1-amine. The marketed form is a partial hydrochloride salt, being present as 40% amine·HCl and 60% sevelamer base. The amine groups of sevelamer become partially protonated in the intestine and interact with phosphorus molecules through ionic and hydrogen bonding.
Structure XIV Sevelamer. The structure shows the exposed binding site for phosphates – the repeating and adjacent NH2 groups
Some other pharmaceutical ion exchangers are listed in Table 7.10.
|
The dose both of calcium salts and of sevelamer is high, being 1.25 g for Calcichew tablets and 800 mg for sevelamer (Renegel; Renvela), and patients who are already on several medications have reported problems with compliance.16 Sevelamer tablets are thus large and one patient reports: ‘the tablets are so disgusting, their consistency is so disgusting – so disgusting that you don’t want to take [them]’. The concept is good with the agents, but the compounds are insufficiently powerful adsorbents or ion-exchangers to allow dosage reduction. Tablets not taken are a negation of therapeutics. What is required are more powerful systems. |
|
Administered orally, cation-exchange resins effect changes in the electrolyte balance of the plasma by exchanging cations with those in the gut lumen. In the ammonium form, cation-exchange resins are used in the treatment of retention oedema and for the control of sodium retention in pregnancy. Depletion of plasma potassium can be prevented by including a proportion of resin in the form of the potassium salt. These resins are also used (as calcium and sodium forms) to treat hyperkalaemia. |
Table 7.10 Ion exchangers used in pharmacy
Name | Type | Comments | Trade name |
Ammonium polystyrene sulfonate | Cation exchanger | Each gram exchanges 2.5 mEq Na+ | Katonium |
Polycarbophil | Cation exchanger | Synthetic hydrophilic resin copolymer of acrylic acid, loosely crosslinked with divinyl glycol. Marked water-binding capacity | – |
Calcium polystyrene sulfonate | Cation exchanger | Each gram exchanges about 1.3 mEq K+ | Calcium Resonium |
Colestyramine | Anion exchanger | The chloride of a strongly basic anion-exchange resin containing quaternary ammonium groups attached to styrene-divinylbenzene copolymer | Questran |
Polyamine–methylene resin | Anion exchanger | Effects a temporary binding of HCl + pepsin in the stomach, later released in the intestine | – |
Sodium polystyrene sulfonate | Cation exchanger | Each gram exchanges 2.8–3.5 mEq K+ | Resonium A |
Polyaminostyrene | Weak anion exchanger |
| – |
Ion-exchange resins are employed to form complexes with drug substances, especially basic drugs such as ephedrine, pholcodine (XV) and phenyltoloxamine (XVI) (an isomer of diphenhydramine) to prolong drug action. Pholtex is a sustained-action liquid utilising a sulfonic acid ion-exchange resin with pholcodine and phenyltoloxamine as resin complexes.
Structure XV Pholcodine
Structure XVI Phenyltoloxamine
The ion-exchange resin (Amberlite IRP-69 resin) has been used with betaxolol as an ophthalmic suspension. This formulation with 0.25% betaxolol gave levels of drug in the aqueous humour virtually identical to those with the 0.5% control.17 The rate of release of basic drugs from cation-exchange resins depends on the diameter of the resin beads, on the degree of crosslinking within the resin, and on the pKa of the ionisable resin group. The resin–drug complex may be tableted and administered orally; resin complexes have been used to mask the taste of bitter drugs and to reduce the nausea produced by some irritant drugs. Some types, such as polacrilin potassium (Amberlite IRP 88, sulfonated polystyrene resin) are used as tablet disintegrants because of the high degree of swelling the dry resins undergo on interaction with water.
Apart from these medical uses, ion-exchange resins are widely used in the removal of ionised impurities from water. Purified water may be obtained by passing through two columns containing a strong cation exchanger and a strong anion exchanger, respectively, or a column containing mixed resins. Anionic impurities in the water are replaced by OH− from the anion exchanger and cations by H+ from the cation-exchange resin. Dissolved salts are thus removed and replaced by H2O molecules, but of course non-ionic impurities and colloidal material are not removed. Regeneration of the resins is accomplished using NaOH and HCl for the anion and cation exchangers, respectively.
7.5.3 Silicone oligomers and polymers
The silicones are examples of hydrophobic liquid polymers, although in high molecular weight they exist as waxes and resins. Silicones are polymers with a structure containing alternate atoms of silicon and oxygen; the dimethicones are fluid polymers with the general formula (XVII), in which each unit has two methyl groups and an oxygen atom attached to the silicon atom in the chain. The viscosity range extends from 0.65 cSt to 3 × 106 cSt. The dimethicones 20, 200, 350 and 1000 (the number representing the average viscosity in centistokes at 25°C) have rheological properties that allow them to be used in ophthalmology and in rheumatoid arthritis. Dimethicone 200 has been used as a lubricant for artificial eyes and to replace the degenerative vitreous fluid in cases of retinal detachment. It can also act as a simple lubricant in joints. More common uses are as barrier substances, silicone lotions and creams acting as water-repellent applications protecting the skin against water-soluble irritants. Methylphenylsilicone is used as a lubricant for hypodermic syringes. Glassware which has been treated with a thin film of silicone is rendered hydrophobic; solutions and aqueous suspensions thus drain completely from such vessels.
Structure XVII Dimethicone
Activated dimethicone (activated polymethylsiloxane) is a mixture of liquid dimethicones containing finely divided silica to enhance the defoaming properties of the silicone. The mechanism by which dispersion of colloidal silica antifoams improves their action is not well understood.
By varying the amounts of polymer in resin, a variety of products such as catheters, tubing materials for reconstructive surgery and membranes for drug reservoirs can be formed.
The release of lipophilic steroids from silicone elastomer matrices is dependent on the crosslinking density of the polymer and the content of filler, but also on the lipophilicity of the drug. A relationship between the solubility parameters of a number of drugs and their release rate is shown in Fig. 7.31.
Figure 7.31 Comparative release rates of different drugs from a pressure-sensitive silicone matrix as a function of their solubility parameter.
Reproduced from Clauss LC. In: Proceedings of the International Conference on Pharmaceutical, Ingredients and Intermediates (1990). Maarssen: Expoconsult; 1991: 116.
An interesting application of the silicone fluids is their coformulation with adhesives to increase ‘tack’ and in elastomers to soften the product. Pressure-sensitive silicone adhesives are formed from the most highly crosslinked systems based on the structure XVII.
Other pressure-sensitive adhesives used in transdermal delivery device include those shown in Table 7.11.
Table 7.11 Pressure-sensitive adhesives in transdermal patches
Adhesive class | Adhesive polymer |
Polyisoprene | |
Ethyl acrylate 2-Ethylhexyl acrylate | |
Polydimethylsiloxane |
Reproduced from Pfister WR et al. Pharm Tech 1992;16(1):42.
|
|
7.6 Some applications of polymeric systems in drug delivery
Control of the rate of release of a drug when administered by oral or parenteral routes is aided by the use of polymers that function as a barrier to drug movement.
7.6.1 Film coating
Aspects of film-coating technology have already been discussed in Chapter 1. Here we concentrate on the polymers used to achieve the desired release properties.
Polymer solutions allowed to evaporate produce polymeric films that can act as protective layers for tablets or granules containing sensitive drug substances or as a rate-controlling barrier to drug release. Film coats have been divided into two types: those that dissolve rapidly and those that dissolve very slowly and behave as dialysis membranes, allowing slow diffusion of solute or some delayed diffusion by acting as gel layers. Materials that have been used as film formers include shellac, zein, glyceryl stearates, paraffins and a range of anionic and cationic polymers such as the Eudragit polymers (XVIII–XXI). Newer materials used for the same purpose include cellulose acetate phthalate (CAP).
Different film coats applied to tablet surfaces can lead to quite different rates of solution. In Fig. 7.32, HPMC, an acrylic derivative, and zein are compared for their effect on sodium chloride dissolution from discs. Two plasticisers have been used: glycerin and diethyl phthalate. Times for 50% dissolution range from a few minutes to 450 minutes, indicating the scope of the technique for retard formulations, and the possibility of unwittingly extending dissolution times by the injudicious choice of coating material.
Figure 7.32 Dissolution of sodium chloride from tablets coated with hydroxypropylmethylcellulose (○), a vinyl polymer (●) and zein (⊙) with glycerin as an additive, and the same polymers with diethyl phthalate as additive (◻, ◼, and ⊡, respectively).
Reproduced from Laguna O et al. Ann Pharm Franc 1975;33:235.
The process of film coating generally involves spraying the chosen polymer solution on to a bed of tablet cores. Several physical properties are important, for example, the viscosity and surface tension of the solutions will determine the droplet size and might also influence the spreading of the polymer on the tablet surfaces as well as its adhesion to the substrate. Additives are used to modify the native properties of the polymer solutions; in one example the addition of microcrystalline cellulose allowed a greater interaction with the tablet surface, probably through interaction with the same material in the tablet formulation. Polymer blends are used to tailor release rates of drugs from coated tablets. As with many pharmaceutical processes, matters are far from simple. Blended polymers may be incompatible, polymer dispersions may flocculate and plasticisers migrate. Ultimately the coated systems must be tested rigorously, with respect to their main functions, control of drug release rate, reduction of water permeability into the core and reduced oxygen permeability to minimise degradation.
Structure XVIII Poly(methacrylic acid, methyl methacrylate) 1 : 1 copolymer (Eudragit L12.5, L100)
Structure XIX Poly(ethylacrylate, methacrylic acid) 1 : 1 (Eudragit L 30 D, L 100–55)
Structure XX Poly(butylmethacrylate, 2-dimethylaminoethyl methacrylate, methyl methacrylate) 1 : 2 : 1 (Eudragit E 100, E 12.5)
Structure XXI Poly(ethylacrylate, methyl methacrylate) 2 : 1 (Eudragit E 30 D)
7.6.2 Matrices
The use of a barrier film coating is only one of several procedures that can be adopted to control release of drugs (Fig. 7.33). Various methods are shown schematically in Table 7.12. If hydrophobic water-insoluble polymers are used, the mechanism of release is the passage of drug through pores in the plastic, or by leaching or slow diffusion of drug through the polymer wall (Fig. 7.33b), as discussed earlier in this chapter. Release may also be effected by erosion of the polymer (Fig. 7.33c). When water-soluble polymers are employed, for example as hydrophilic matrices, the entry of water into the polymer is followed by swelling and gelation and the drug must diffuse through the viscous gel, a process obviously slower than diffusion through plain solvent.
Table 7.12 Depot forms employing polymeric films and matrices
Type | Materialsa | Diagrammatic representation | Mechanisms |
Barrier coating | Beeswax, glyceryl monostearate, ethylcellulose, nylon (Ultramid IC), acrylic resins (Eudragit retard) | Diffusion | |
Fat embedment | Glycerol palmitostearate (Precirol), beeswax, glycowax, castorwax, aluminium monostearate, carnauba wax, glyceryl monostearate, stearyl alcohol | Erosion, hydrolysis of fat, dissolution | |
Plastic matrix | Polyethylene | Leaching, diffusion | |
Repeat action | Cellulose acetylphthalate | Dissolution of enteric coat | |
Ion exchange | Amberlite |
| Dissociation of drug–resin complex |
Hydrophilic matrix | Carboxymethylcellulose | Gelation, diffusion | |
Epoxy resin beads | Epoxy resins | Dissolution of resin or swelling, diffusion | |
Microcapsules | Polyamides, gelatin |
|
|
Soft gelatin depot capsules | Shellac–PEG |
| Diffusion |
Modified from Ritschel WA. In: Ariens AJ (ed.) Drug Design, vol. IV. New York: Academic Press; 1974, with permission.
aMaterials used are not all polymeric. The waxes are included for completeness; these depend on conferring a hydrophobic layer on the drug, tablet or granule to prevent access of solvent.
Figure 7.33 (a) Drug delivery from a matrix delivery device showing diagrammatically the depletion of drug from the system and the release of the drug. (b) Release from a typical reservoir system with a membrane controlling release from the internal store of drug. (c) Illustrating release from (i) a bulk-eroding system and (ii) a surface-eroding system.
After Brandon-Peppas L. Polymers in controlled drug delivery. Med Plast Biomaterials 1997;Nov.: 34, with permission.
Release of drugs from matrices
Equations describing the rate of drug release from hydrophobic and hydrophilic matrices are useful in determining which factors may be altered to change the measured release rate of drug. Higuchi18 proposed the following equation for the amount of drug, Q, released per unit area of tablet surface in time t, from an insoluble matrix:
D is the diffusion coefficient of the drug in the release medium, Cs is the solubility of drug in the medium, ε is the porosity of the matrix, τ is the tortuosity of the matrix and A is the total amount of drug in the matrix per unit volume.
If the same matrix is saturated with a solution of the drug (as in medicated soft contact lenses) the appropriate equation becomes, if C0 is the concentration of drug solution:
That is, for a given drug in a given matrix, Q t1/2. The more porous the matrix, the more rapid the release. The more tortuous the pores, the longer the path for diffusing molecules, the lower is Q. More soluble drugs diffuse more quickly from the matrix.
The extension of these equations to hydrophilic matrices is difficult because the conditions in a hydrophilic matrix change with time as water penetrates into it. If the polymer does not dissolve but simply swells and if the drug has not completely dissolved in the incoming solvent, diffusion of drug commences from a saturated solution through the gel layer, and
Equation (7.28) is similar to equation (7.26) except that the effective volume V of the hydrated matrix is used as this is not a fixed quantity. W0 is the dose of the drug in the matrix. If the drug completely dissolves on hydration of the matrix, an analogue of equation (7.27) is used:
In the initial stages of the process the rate of movement of water into the matrix may be important in determining release characteristics. When a homogeneous barrier wall is present diffusion through the walls has to take place and equations in section 7.5.1 apply.
7.6.3 Microcapsules and microspheres
Microencapsulation is a technique that, as its name suggests, involves the encapsulation of small particles of drug, or solution of drug, within a polymer film or coat. Microspheres, on the other hand, are solid but not necessarily homogeneous particles that can entrap drug. Although the terms tend to be used interchangeably, we retain the distinction here. Microspheres can be prepared also by a variety of techniques, discussed briefly below. Typical photomicrographs of poly(ε-caprolactone) microspheres are shown in Fig. 7.34. Three main processes can be considered:
1. Coacervation of macromolecules around the core material, this being induced by temperature change, solvent change or addition of a second macromolecule of appropriate physical properties.
2. Interfacial polymerisation of a monomer around the core material by polymerisation at the interface of a liquid dispersion.
3. Spray coating and other methods in which larger particles may be coated in suspension.
Figure 7.34 Scanning electron micrographs of bovine serum albumin-loaded microspheres prepared from a ternary blend of poly(ε-caprolactone). Protein entrapment efficiency and mean particle size were 28.6% and 2.9 μm, respectively.
Reproduced with permission from Huatan H et al. The microencapsulation of protein using a novel ternary blend based on poly (caprolactone). J Microencapsul 1995;12:557.
Any method that will cause a coherent barrier to deposit itself on the surface of a liquid droplet or a solid particle of drug may be applied to the formation of microcapsules. Many so-called microencapsulation procedures result in the formation of macroscopic ‘beads’, which are simply coated granules.
Coacervation
Coacervation is the term used to describe the separation of macromolecular solutions into colloid-poor and colloid-rich (coacervate) phases when the macromolecules are desolvated. The liquid or solid to be encapsulated is dispersed in a solution of a macromolecule (such as gelatin, gum arabic, carboxymethylcellulose or poly(vinyl alcohol)) in which it is immiscible. A non-solvent, miscible with the continuous phase but a poor solvent for the polymer, under certain conditions will induce the polymer to form a coacervate (polymer-rich) layer around the disperse phase. This coating layer may then be treated to give a rigid coat of capsule wall. This is the process of simple coacervation. Successful application of the technique relies on the determination of the appropriate conditions for coacervate deposition, which can be achieved not only by the addition of non-solvents such as ethanol and isopropanol and salts (sodium and ammonium sulfates) but also by the choice of macromolecules incompatible under selected conditions with the first species. The latter process is termed complex coacervation. In both simple and complex coacervation utilising hydrophilic macromolecules it is the decrease in solubility that results in deposition of the macromolecule layer at the particle–solution interface.
Desolvation of water-insoluble macromolecules in non-aqueous solvents leads to the deposition of a coacervate layer around aqueous or solid disperse droplets. Table 7.13 lists both water-soluble and water-soluble macromolecules that have been used in coacervation processes. Desolvation, and thus coacervation, can be induced thermally and this is the basis of some preparative techniques. Conditions for phase separation are best obtained using phase diagrams.
Table 7.13 Materials used in coacervation microencapsulation
Water-soluble macromolecules | Water-insoluble macromolecules |
Arabinogalactan | Cellulose acetate phthalate |
Carboxymethylcellulose | Cellulose nitrate |
Gelatin | Ethylcellulose |
Gum arabic (acacia) | Poly(ethylene vinyl acetate) |
Hydroxyethylcellulose | Poly(methyl methacrylate) |
Poly(acrylic acid) |
|
Polyethyleneimine |
|
Poly(vinyl alcohol) |
|
Polyvinylpyrrolidone |
|
Methylcellulose |
|
Starch |
|
For example, in the region of pH 6, gelatin will be positively charged and gum arabic negatively charged. In admixture, complex coacervates will form under the conditions described in Fig. 7.35, which shows the partial phase diagram of the ternary system gum arabic–gelatin–water. The hatched area at the top of the phase diagram is the restricted region in which coacervation occurs. At higher concentrations of the macromolecules, macroscopic precipitates of the complex will occur.
Figure 7.35 Ternary diagram showing complex coacervation region for mixtures of gum arabic and gelatin at pH 4.5; below the curved line the mixture separates into two sols (US Patent 2800457). Insert: Typical droplets formed by coacervation (Ronald T Dodge).
Interfacial reaction
Reactions between oil-soluble monomers and water-soluble monomers at the oil–water interface of water-in-oil (w/o) or o/w dispersions can lead to interfacial polymerisation, resulting in the formation of polymeric microcapsules, the size of which is determined by the size of the emulsion droplets. Alternatively, reactive monomer can be dispersed in one of the phases and induced to polymerise at the interface, or to polymerise in the bulk disperse phase and to precipitate at the interface owing to its insolubility in the continuous phase. There are many variations on this theme. Probably the most widely studied reaction has been the interfacial condensation of water-soluble alkyl diamines with oil-soluble acid dichlorides to form polyamides. Representative examples of other wall materials are polyurethanes, polysulfonamides, polyphthalamides and poly(phenyl esters). The selection of polymer is restricted to those that can be formed from monomers with the requisite preferential solubilities in one phase so that polymerisation takes place only at the interface.
Physical methods of encapsulation
Various physical methods of preparing microcapsules such as spray drying and pan coating are available.
Pan-coating application of films to particles can only be used for particles greater than 600 μm in diameter. The process has been applied in the formation of sustained-release beads by application of waxes such as glyceryl monostearate in organic solution to granules of drug.
The spray-drying process involves dispersion of the core material in a solution of coating substance and spraying the mixture into an environment that causes the solvent to evaporate. In an analogous spray-congealing process the coating material is congealed thermally or by introducing the core coat mixture into a non-solvent mixture. Both processes can produce microcapsules in the size range 5000–6000 μm.
Spray polycondensation is a variant based on the polycondensation of surface-active monomers on a melamine–formaldehyde base on the surface of suspended particles during spray drying. A dispersion of the core material is prepared in a continuous phase containing aminoplast monomers or precondensates of relatively low molecular weight, in addition to other film-forming agents and catalyst. The reactive monomers derived from hexamethylol melamine derivatives are selectively adsorbed at the surface of the disperse phase. Spray drying at 150–200°C results in vaporisation of the water and causes simultaneous polycondensation of the monomers and precondensates by acid catalysis.
The preparation and properties of nanoparticles for drug delivery and targeting are discussed in Chapter 14.
General considerations
In all techniques discussed there are several factors which are of importance in relation to the use of the product as a drug delivery vehicle or carrier.
- the efficiency of encapsulation of the active ingredient
- the effect of the encapsulation process on the properties of the encapsulated agent
- the presence of potentially toxic residue (e.g. monomer, salts) in the final product
- the reproducibility of the process and ease of separation from the reaction mixture
- the biocompatibility of the encapsulating agent
- the biodegradability of the material (in some cases)
- the properties of the microcapsule in relation to use, size distribution, porosity and permeability of the wall.
Biodegradable polymers cannot always be induced to form microcapsules and a polymer found to be degradable in solid or film form may not be degradable when formed into microcapsules if the polymer chains are crosslinked. In their application in medicine, the permeability of the capsule wall is probably the most important feature of the product. The effects of the various parameters relating to wall material, capsule and environment on permeability are outlined in Table 7.14.
Table 7.14 General parameters affecting capsule wall permeability
Parameter | For lower permeability |
Properties of wall polymer | |
Density | Increase |
Crystallinity | Increase |
Crosslinking | Increase |
Plasticiser content | Decrease |
Fillers | Increase |
Solvents used in film formation | Use good solvents versus poor |
Properties of capsule | |
Size | Increase |
Wall thickness | Increase |
Treatment | Utilise (e.g. crosslinking, sintering) |
Multiple coatings | Utilise |
Environmental properties | |
Temperature | Decrease |
Reproduced with permission from Vandegaer JE (ed.) Microencapsulation, Processes and Applications. New York: Plenum Press; 1974.
Protein microspheres
Aqueous solutions of proteins such as albumin can be emulsified in an oil and induced to form microspheres, either by crosslinking the protein molecules with glutaraldehyde or other agents or by coagulating the protein by heating. Incorporation of a drug within the initial protein solution results in drug-laden microspheres that are biodegradable. The particle size of the microspheres (generally 0.2–300 μm diameter) is determined by the size distribution of the initial emulsion.
Protein microspheres have been used for physical drug targeting, i.e. the entrapment of carrier and therefore drug in, for example, the capillaries of the lung. Microspheres greater than about 7 μm in diameter will be physically trapped in capillary beds. On intra-arterial injection of large microspheres, the blood supply to an organ is reduced; the process of chemoembolisation involves both blockage and delivery of drugs to the organ. External control over intravenously administered protein microspheres has been achieved by incorporation of magnetite (Fe3O4) particles into the microspheres, which then respond to an externally applied magnetic field.
7.6.4 Rate-limiting membranes and devices
The use of rate-limiting membranes to control the movement of drugs from a reservoir has been referred to above. Implants of silicone rubber or other appropriate polymeric material in which drug is embedded can be designed by choice of polymer, membrane thickness and porosity, to release drug at preselected rates. The Progestasert device (Fig. 7.36c) was designed to be implanted into the uterine cavity and to release there 65 μg progesterone per day to provide contraceptive cover for 1 year. Progestasert was discontinued in 2001, but similar devices such as Mirena (Bayer) are available to deliver levonorgestrel. The Ocusert device and the Transiderm therapeutic system (also shown in Fig. 7.36) originated from the then Alza Corporation (USA) and rely on rate-limiting polymeric membranes to control drug release. The opportunities for prolonged release of drugs given by the oral route are fewer. The aim in oral dosage forms is for controlled rather than prolonged release, so that dosage frequency can be reduced or so that side-effects resulting from fast dissolution of drug can be minimised.
Figure 7.36 Examples of iconic drug delivery systems employing polymeric membranes. (a) Ocusert system for the eye with two rate-controlling membranes. (b) Transiderm system for transdermal medication with one rate-controlling layer. (c) The Progestasert device for intrauterine insertion in which the body of the device serves as the rate-controlling barrier. (d) The oral Oros device, in which the membrane is a semipermeable membrane that prevents drug transport, allowing water ingress only.
7.6.5 Eroding systems
Release of drug by erosion of the polymeric or macromolecular matrix in which a drug is dissolved or dispersed provides another mechanism for controlling drug absorption. Erosion, of course, demands some degree of solubility of the matrix to allow enzymes and other agents to penetrate. A typical bioerodible system would be that achieved by the molecular association of a CAP (a carboxylic acid polymer) with a poloxamer block copolymer such as Pluronic L101. This interaction is between the proton-donating CAP and the proton-accepting poloxamer. By varying the ratio of CAP to poloxamer, the erosion periods can be controlled from hours to days. Figure 7.37 shows the relationship between the percentage polymer eroded and the release of drugs from a 50 : 50 mixture of the polymers containing 10% of the drug metronidazole.
Figure 7.37 Characteristics of in vivo polymer erosion and metronidazole (MTZ) release from 50/50 CAP/Pluronic L101 films (with 10% metronidazole loading) in a dorsal rat model.
Reproduced from Gates KA et al. A new bioerodible polymer insert for the controlled release of metronidazole. Pharm Res 1994;11:1605, with kind permission from Springer Science and Business Media.
Computer simulation of eroding matrices (Fig. 7.38) can give an accurate representation of the process and can predict the position of the erosion front and the weight of the matrix.
Figure 7.38 Theoretical representation of a polymer matrix: changes during erosion (dark pixels = non-degraded areas, white pixels = degraded areas), where λa (a rate constant) = 2.7 × 10−7 s−1 for a sample containing 50% polyanhydride 1-3 bis(pcarboxyphenoxypropane).
Reprinted with permission from Göpferich A et al. In: Cleland JL, Langer R (eds) Formulation and Delivery of Proteins and Peptides. ACS Symposium Series no. 567. Washington, DC: American Chemical Society; 1994. Copyright 1994 American Chemical Society.
More precise control of release than is possible with matrices has recently been achieved by the application of several features of polymer physical chemistry, as discussed below.
7.6.6 Osmotic pumps
A variety of osmotic pumps have been described in the patent literature.19,20 In the oral osmotic pump (Oros or Osmet) the drug is mixed with a water-soluble core material. This core is surrounded by a water-insoluble semipermeable polymer membrane in which is drilled a small orifice. Water molecules can diffuse into the core through the outer membrane to form a concentrated solution inside. An osmotic gradient is set up across the semipermeable membrane, with the result that drug is pushed out of the orifice (Fig. 7.39).
Figure 7.39 Low-power scanning electron micrographs of an oral osmotic pump (Osmet). (a) A section showing the semipermeable membrane, the osmotic core and the laser-drilled orifice. (b) The same, 8 hours after immersion in water. (c) The laser-drilled orifice. (d) Another view of the tablet via the orifice after 24 hours of immersion.
The core may be a water-soluble polymer, an inert salt or, as in the case of metoprolol fumarate,21 the drug itself, whose saturated solution has an osmotic pressure of 32.5 atm. The osmotic tablet of nifedipine is described in detail in Fig. 7.40, which shows the semipermeable cellulose acetate coating, the swellable hydrogel layer of PEG and HPMC and the drug chamber containing nifedipine in HPMC and PEG.
Figure 7.40 Cross-section of the ‘push–pull’ osmotic pump for nifedipine.
Reproduced from Zaffaroni A. Overview and evolution of therapeutic systems. Ann N Y Acad Sci 1991;618:405. Copyright Wiley-VCH Verlag GmbH & Co. KGaA. Reproduced with permission.
For simple osmotic systems the initial zero-order delivery rate (dm/dt) is given by
where Sd is drug concentration in the system, πf is the osmotic pressure of the formulation, πe is the osmotic pressure of the environment (7.7 atm for isotonic saline at 37°C), k is the membrane permeability to water, H is the thickness of the membrane and A is its area. Calculated and experimental release rates are shown in Fig. 7.41.
Figure 7.41 In vitro release rate of potassium chloride from elementary osmotic pumps in water at 37°C.
Reproduced from Theeuwes F. Elementary osmotic pump. J Pharm Sci 1975;64:1987. Copyright Wiley-VCH Verlag GmbH & Co. KGaA. Reproduced with permission.
One problem is that of controlling the transit of the device down the gastrointestinal tract, as individual subjects vary considerably in gastrointestinal transit times. If the system is designed to release drug over a period of 10 hours and total transit time in the gut is 5 hours, bioavailability will obviously be reduced.
7.6.7 Transdermal delivery systems
Several transdermal systems ostensibly dependent on rate-controlling membranes are available for the delivery of glyceryl trinitrate, hyoscine, estradiol, fentanyl and other drugs. The word ‘ostensibly’ is used as there is a debate about whether the barrier membrane in these devices is the rate-limiting step in absorption. The barrier properties of skin are so variable, however, that one advantage of rate-controlling systems is that they prevent too rapid dosing in patients with highly permeable skin. In those with less permeable skin, the systems probably act only as reservoirs. The wide range of agents listed in Table 7.15, has been supplemented by buprenorphine, testosterone, selegiline and 5-hydroxytryptophan among others.
Table 7.15 Some transdermal drug delivery devices
Product | Drug | Adhesive | Adhesive usea | Marketing company |
Antianginal | ||||
Nitrodisc | GTNb | Acrylic | Rim | G. D. Searle |
Nitro-Dur I | GTN | Acrylic | Rim | Schering-Plough |
Nitro-Dur II | GTN | Acrylic | Matrix | Schering-Plough |
Diafusor | GTN | Acrylic | Matrix | Lab Pierre Fabre Med |
Minitran | GTN | Acrylic | Matrix | 3M Riker |
Transiderm-Nitro | GTN | Silicone | Face | Novartis |
Nitroderm TTS | GTN | Silicone | Face | Novartis |
Nitrol patch | GTN | Acrylic | Rim | Adria Lab. |
NTS patch | GTN | Acrylic | Rim | Bolar, Major, Qualitest, Bio-Line, Goldline, Geneva, Rugby |
Transdermal-NTG | GTN | Acrylic | Rim | Warner Chilcott Lab. |
Nitrocine | GTN | Acrylic | Rim | Kremer Urban |
Deponit | GTN | Polyisobutylene | Matrix | Schwarz/Wyeth |
Frandol Tape | Isosorbide dinitrate | Acrylic | Matrix | Toaeiyo, Yamanouchi Pharm. |
Motion sickness | ||||
Trasiderm-Scop | Hyoscine | Polyisobutylene | Matrix | Novartis |
Kimite-patch | Hyoscine | Polyisobutylene | Matrix | Myun Moon Pharm. Co. |
Hypertension | ||||
Catapress-TTS | Clonidine | Polyisobutylene | Matrix | Boehringer Ingelheim |
Oestrogen therapy | ||||
Estraderm | Estradiol | Polyisobutylene | Face | Novartis |
Analgesia | ||||
Duragesic | Fentanyl | Silicone | Face | Jansen Pharm. |
Smoking cessation | ||||
Nicotinell | Nicotine | Acrylic | Matrix | Novartis |
Nikofrenon | Nicotine | Acrylic | Matrix | Novartis |
Reproduced with permission from Foster WP et al. Pharm Tech 1992;16(1):42.
aIndicated as an adhesive laminated to an overlying backing substrate forming a rim around the matrix (rim): a drug-containing adhesive matrix laminated to a backing substrate (matrix); or an adhesive laminated to the face of a rate-controlling membrane (face).
bGTN = glyceryl trinitrate.
Some of the devices and the bases of their design are shown in Fig. 7.42 (the Transiderm system is also shown in Fig. 7.36). There are two groups: membrane and matrix systems. Membrane systems generally consist of a reservoir, a rate-controlling membrane and an adhesive layer. Diffusion of the active principle through the controlling membrane governs release rate. The active principle is usually present in suspended form; liquids and gels are used as dispersion media. In matrix systems the active principle is dispersed in a matrix that consists either of a gel or of an adhesive film.
Figure 7.42 The structures of some commercial transdermal membrane-controlled and matrix systems (the structure of the Deponit TTS is shown in Fig. 7.43).
In Transiderm Nitro, the rate-controlling membrane is composed of a polyethylene–vinyl acetate copolymer having a thin adhesive layer (membrane type) (Table 7.15). The reservoir contains glyceryl trinitrate dispersed in the form of a lactose suspension in silicone oil. The Nitro-Dur system consists of a hydrogel matrix (composed of water, glycerin, poly(vinyl alcohol) and polyvinylpyrrolidone) in which a glyceryl trinitrate–lactose triturate is homogeneously dispersed.
In Nitro Disc, glyceryl trinitrate is distributed between microscopically small liquid compartments and a crosslinked silicone matrix. In the approximately 10–200 μm sized micro compartments there is active ingredient also in the form of a lactose triturate, in an aqueous solution of PEG 400. The system is secured to the skin with the aid of a circular adhesive disc having a centrally located silicone matrix, not coated with adhesive. The system is termed a ‘micro sealed drug delivery system’. The basis of the Deponit system22 is shown in detail in Fig. 7.43.
Figure 7.43 Cross-section of Deponit TTS. Deponit contains glyceryl trinitrate directly embedded in a lactose-containing adhesive film approximately 0.3 mm thick. In cross-section, the Deponit transdermal system, closely resembling a plaster, consists of three components in macroscopic proportions, the ratios of which are as given in the figure. The adhesive film is both a store of active ingredient and the release-controlling matrix.
Reproduced from Wolff M et al. In vitro and in vivo release of nitroglycerin from a new transdermal therapeutic system. Pharm Res 1985;2:23–29, with kind permission from Springer Science and Business Media.
|
|
7.6.8 Polymer fibres: electrospinning
We have discussed polymers as thin films, matrices, microspheres and nanospheres. In this section we treat briefly the concepts of the formation of polymer fibres which are being increasingly studied as delivery devices, or as materials for wound dressings, and transdermal delivery systems.
Electrospinning is an electrohydrodynamic process which uses electrostatic forces to drive fibres (or droplets) from a capillary via an electrically charged fluid jet. The topic of electrospinning has a long pedigree, dating from experiments in the 16th century when experiments were carried out on the effect of electric potential on beeswax and shellac in liquid form. As the name suggests, the phenomenon relates to the effect of electrostatic forces on a liquid droplet, a topic developed theoretically to understand the formation of Taylor cones (Fig. 7.44) and filaments which, if a polymer solution is in the capillary and the applied field is sufficiently strong, will be the basis of polymer fibres.
Figure 7.44 Left: The formation of a Taylor cone, showing the effect of the application of a high voltage to a needle tip, causing surface charges to accumulate on the liquid, leading to the deformation of the spherical drop into a cone. Increase in the voltage beyond this level causes the formation of a liquid jet. Right: The forces acting on the system which shape the nature of the jet include surface tension, viscous forces and normal and tangential electrical stresses.
When an appropriately high voltage is applied to a liquid droplet, the liquid becomes charged, and electrostatic repulsion counteracts the surface tension; the droplet is pulled out or stretched and at a critical point a stream (jet) of liquid erupts from the surface. This point is known as Taylor cone formation. As the jet travels towards the collector, tensile forces brought about by surface charge repulsion lead to a bending motion and the polymer chains in the jet orient to form microfibres or nanofibres depending on the polymer, its viscosity, surface tension and the capillary diameter; the polymer is then deposited on an earthed collector.
The current interest in the technique is that it allows the formation of fibres with a variety of internal structures and dimensions, these having potential for drug delivery and the application of drugs in novel ways. Some of these structures are shown in composite micrographs in Fig. 7.45.
Figure 7.45 Photomicrographs of several fibrous structures prepared by electrospinning of polymers.
Reproduced from Chakraborty S et al. Electrohydrodynamics: a facile technique to fabricate drug delivery systems. Adv Drug Deliv Rev 2009;61:1043. Copyright Elsevier 2009.
A typical small-scale manufacturing device is shown in Fig. 7.46, which shows both monoaxial and coaxial processes, the former producing mainly monolithic fibres and the latter core-shell structures. Drug can be incorporated into the polymer (or indeed ceramic) materials to provide interesting geometries which can be manipulated for drug delivery.
Figure 7.46 Two devices for electrospinning polymer solutions: (a) a monoaxial system in which a solution containing drug flows through a capillary to which a voltage is applied to form a monolithic fibre; and (b) the coaxial system in which the polymer can be made to surround a core system by the expedient of using a core drug polymer and a shell polymer solution. In both cases a collector which is grounded receives the fibres.
Reproduced from Chakraborty S et al. Electrohydrodynamics: a facile technique to fabricate drug delivery systems. Adv Drug Deliv Rev 2009;61:1043–1054. Copyright Elsevier 2009.
What factors control the nature of the product? Those listed in Fig. 7.47 can be rationalised readily, large-diameter fibres being produced by increasing polymer concentration, increased molecular weight of the polymers, enhanced flow rate, increased needle diameter and increased viscosity of the solutions used. Smaller-diameter fibres form with increased field strength, increased conductivity of the solutions, enhanced solvent volatility, increased surface tension and the distance to the collector, as shown in Fig. 7.43. The physics is quite complex; however, it is important to know in more detail the outlines of the parameters concerned. The diameter of the fluid jet, d, is related to the flow rate, Q, of the fluid, the fluid density, ρ, and the applied field strength, E∞, by the equation
where z is an axial coordinate. The value of d determines the diameter of the fibre.
Figure 7.47 Factors affecting the diameter of the fibres produced by electrospinning.
Reproduced from Chakraborty S et al. Electrohydrodynamics: a facile technique to fabricate drug delivery systems. Adv Drug Deliv Rev 2009;61:1043–1054. Copyright Elsevier 2009.
7.6.9 Polymers in wound dressings
The treatment of wounds is a complex topic, such are the variety of skin lesions and the types of wound dressings now available to aid the healing process. As many dressings involve synthetic or natural polymers and macromolecules and rely for some of their benefits on physical phenomena such as capillarity and wicking, it is appropriate to discuss these systems here. One of the purposes of a dressing is to keep the wound moist, to absorb exudate and to maintain gas exchange. The British National Formulary 68 states that ‘advanced wound dressings’ are designed to control the environment for wound healing, for example, to donate fluid (e.g. with hydrogels), maintain hydration (e.g. hydrocolloids) or to absorb wound exudate (e.g. alginates, foams).
Wound dressings can generally be classified23 in groups depending on their nature and properties, e.g.:
- semipermeable films
- hydrocolloids
- alginates
- polymer foams
- hydrogels
- antimicrobial-releasing dressings.
There are naturally some overlaps in definitions and structures. We discuss a selection of structures here to illustrate their nature and variety.
Hydrocolloids
Hydrocolloids are composed of gel-forming substances such as gelatin or NaCMC. One product, Hydrofiber, is an NaCMC dressing with a high cohesive dry and wet tensile strength which absorbs up to 25 times its weight in fluid without losing its integrity. NaCMC is spun into fibres (see also alginates, below) and is produced in ribbon and sheet formats.
Alginates
Alginates derived from seaweeds have been used pharmaceutically for many years. The alginates which are polyelectrolytes – composed of two sugars, L-glucuronic acid and D-mannuronic acid – form highly absorbent strong gels, which inter alia minimise bacterial contamination. Sodium alginates are soluble, but binding to calcium results in hydrated poorly soluble systems in the form of gels, beads, films and fibres. Calcium alginate fibres are made by extruding sodium alginate solutions through spinnerets into calcium-containing solutions where the fibres precipitate. This approach can be used to prepare zinc alginate and silver alginate fibres.
Foam dressings
Polyurethane is most commonly used to prepare foam dressings. Synthaderm is a ‘synthetic skin’ of a modified polyurethane foam, hydrophilic on one side and hydrophobic on the other (Fig. 7.48). The hydrophilic side is placed in contact with the wound. The system24: (a) maintains a high humidity at the dressing interface; (b) removes excess exudate; (c) allows gaseous exchange; and (d) provides insulation; moreover, it is impermeable to bacteria, the outer surface remaining dry, unlike some saturable dressings.
Figure 7.48 (a) Polyurethane foam and (b) the molecular structure of polyurethane.
(a) Reproduced from www.centexbel.be, with permission.
Lyofoam is a similar product25 – a soft, hydrophobic, polyurethane foam sheet 8 mm thick. The side of the dressing that is to be placed in contact with the skin or wound has been heat-treated to collapse the cells of the foam, and thus enable it to absorb liquid by capillarity. The dressing is freely permeable to gases and water vapour but resists the penetration of aqueous solutions and wound exudate. In use, the dressing absorbs blood or other tissue fluids, and the aqueous component is lost by evaporation through the back of the dressing.
Hydrogels
Hydrogel dressings are most commonly able to conform to the shape of a wound. A secondary, non-absorbent dressing is needed. These dressings are generally used to donate liquid to dry sloughy wounds and facilitate debridement of necrotic tissue; some also have the ability to absorb very small amounts of exudate. Hydrogel sheets have a fixed structure and limited fluid-handling capacity.
Crosslinked dextran gels are insoluble hydrophilic gels that can be partially depolymerised to the required molecular weight. The gel is produced in a bead shape; the degree of crosslinking determines the water uptake and pore size. Dextranomer (Debrisan) is a crosslinked dextran with pores large enough to allow substances with a molecular weight of less than 1000 Da to enter the beads. Each gram of beads abstracts approximately 4 cm3 of fluid. Applied to the surface of secreting wounds, dextranomer removes by suction various exudates that tend to impede tissue repair, while leaving behind high-molecular-weight materials such as plasma proteins and fibrinogen.
Antimicrobial-releasing dressings
Dressings containing antimicrobials such as polihexanide (polyhexamethylene biguanide) or dialkylcarbamoyl chloride are available for use on infected wounds (see British National Formulary). Cadexomer is a dextrin derivative which forms a complex with iodine, cadexomer–iodine, which, like povidone–iodine, releases free iodine when it is exposed to wound exudate. The free iodine acts as an antiseptic on the wound surface; the cadexomer particles embedded in the dressing structure also absorb wound exudate and encourage de-sloughing.
Silver bonded to alginates can form antimicrobial dressings and work as shown in Figure 7.49.
Figure 7.49 Representation of a non-woven alginate dressing with silver in contact with a wound exudate containing bacteria, which are trapped in the swollen alginate as the fibres expand where the released silver then acts.
Reproduced from Qin Y. Silver-containing alginate fibres and dressings. Int Wound J 2005;2:172–176. Copyright Wiley-VCH Verlag GmbH & Co. KGaA. Reproduced with permission.
Wicking
Wicking describes the cumulative action of capillarity in drawing liquid into matrices. In this section we refer to wicking as the process whereby moisture is moved by capillary action from the inside (wound-facing) to the external surface of a dressing. Figure 7.50 reminds us pictorially and graphically of the effect of the diameter of channels on the extent of capillary rise and the fact that channels, especially in fibres and composites, may be asymmetric.
Figure 7.50 From left to right: the effect of capillary action on the liquid rise via capillaries with channels of different diameters; a graph showing calculated water heights for one system as a function of capillary diameter; and a more complex liquid pathway.
To be absorbed in this way the fibres or other structures of the dressing must be wetted by the liquid. The last diagram of Fig. 7.50 shows an uneven channel that will occur in real systems. The Lucas–Washburn equation describes capillary flow in a bundle of parallel cylindrical tubes as one would find in several dressing structures. It provides the relationship between the time, t, it takes a liquid with surface tension, γ, and viscosity, , to travel the distance L in a capillary whose diameter is D
Not surprisingly, various substrates, e.g. packed beds, fibre mats or foams, may show different wicking behaviours due to the shape and dimensions of their flow paths.
When a dry porous medium is brought into contact with a liquid, it will start to absorb the liquid at a rate which decreases over time (Fig. 7.51). For a bar of material with cross-sectional area, A, that is wetted on one end, the cumulative volume, V, of absorbed liquid after a time, t, is
where S is the sorptivity of the medium (in m s−1/2 or mm min−1/2).
The quantity i =V/A (which has the dimension of length) is called the cumulative liquid intake. The wetted length, x, of the bar, that is, the distance between the wetted end of the bar and the so-called wet front, is dependent on the fraction, f, of the volume occupied by liquid. This number f is the porosity of the medium; the wetted length is then
Some authors use the quantity S/f as the sorptivity. The above description is for the case where gravity and evaporation do not play a role.
Figure 7.51 Fluid uptake as a function of time in two experiments with crosslinked dextran gels , the upper plot showing the uptake data as a function of time when the system is covered by an impermeable strip, whereas the lower shows the results when the layer is applied 3 h after surgery. Amongst other things the results demonstrate the importance of the mode of application and the use of backing films.
After Wang PY. Clin Mater 1987;2:91–102.
Capillary-action dressings act by a wicking process. These dressings consist of an absorbent core of hydrophilic fibres sandwiched between two low-adherent wound-contact layers to ensure no fibres are shed on to the wound surface. Wound exudate is taken up by the dressing and retained within the highly absorbent central layer. Some examples of wound dressing structures are described below.
Wound-dressing structures: Vacutex, Cerdak and Mextra
The technologies now involved have increased the efficacy of wound products. Figure 7.52 demonstrates some of these developments in describing several commercial products, Vacutex, Cerdak and Mextra. The process of capillary transport of the liquid exudate (including bacteria) is described in the previous section.
(a) Vacutex
(b) Cerdak
(c) Mextra, a ‘superabsorbent’ dressing
Figure 7.52 (a) Liquid removal by capillary action of fluid from the wound, first by vertical wicking and then by lateral (horizontal) wicking action; (b) to act as a reservoir for the liquid porous ceramic spheres are incorporated into one layer to aid also the extraction process; (c) a four-layer design, showing the wound contact layer, the liquid distribution layer, the absorbent layer and a fluid-repellent backing layer.
Bacterial uptake into dressings along with fluid ingress will depend on the characteristics of the dressing and the nature of the bacteria concerned. Not surprisingly, the transport of bacteria in porous media varies with the size and shape of the organism as well as the nature of the dressing.
Summary
Although there is no strict boundary line, we have divided polymers into water-soluble polymers and water-insoluble systems, typified respectively by materials used to prepare viscous solutions and those that function as barrier membranes or containers. In the first case we have considered the factors controlling their properties: the influence of molecular weight (distribution), branching, charge, flexibility, ionic strength and pH on solution properties. In the case of water-soluble polymers, the main concern has been with solute transport through the polymer bulk. Equations dealing with viscosity and with diffusion have been cited. The variety of pharmaceutical uses of polymers has been described, and the variety of morphologies that polymers can adopt has been emphasised: solutions, gels, microcrystals, crystals, fibres and dendrimers.
When a polymer is being characterised for pharmaceutical use, therefore, much more than its molecular weight distribution should be determined. Its end use will, of course, determine the tests to be applied. The sheer versatility of polymers and the variety of chemical structures and physical forms they can adopt open up fascinating possibilities for the development of novel delivery systems, from nanoparticles (discussed in Chapter 14) to macroscopic and microscopic systems with biodegradable, biocompatible, erodible, leachable, hydrophobic, hydrophilic and all the other characteristics we have discussed in this chapter, which lead one to conclude that their utility is limited only by our ingenuity.
References
1. Svenson S, Tomalia DA. Dendrimers in biomedical applications – reflecting on the field. Adv Drug Deliv Rev 2005;57:2106–2129.
2. Menoge AR et al. Dendrimer based drug and imaging conjugates: design considerations for nanomedical applications. Drug Discov Today 2010;15:171–185.
3. McCahon R, Hardman J. Pharmacology of plasma expanders. Anesth Intensive Care Med 2007;879–881.
4. James WP et al. Calcium binding by dietary fiber. Lancet 1978;1:638–639.
5. Peppas NA, Khare AR. Preparation, structure and diffusional behavior of hydrogels in controlled release. Adv Drug Deliv Rev 1993;1:11–35.
6. Chaubal M. Using chitosan as an excipient for oral drug delivery. Drug Deliv Technol 2003;3:32–34, 36.
7. Lueßen HL et al. Bioadhesive polymers for the peroral delivery of peptide drugs. J Control Release 1994;29:329–338.
8. Hammer ME, Burch TG. Viscous corneal protection by sodium hyaluronate, chondroitin sulfate and methylcellulose. Invest Ophthalmol Vis Sci 1984;25:1329–1332.
9. Tabbara KF, Sharara N. Dry eye syndrome. Drugs Today 1998;34:447.
10. Holly FJ. Formation and rupture of the tear film. Exp Eye Res 1973;15:515–525.
11. Oates KMN et al. Rheopexy of synovial fluid and protein aggregation. Interface 2006;3:164–174.
12. Aviad AD, Houpt JD. The molecular weight of therapeutic hyaluronan (sodium hyaluronate): how significant is it? J Rheumatol 1994;21:297–301.
13. Mori S et al. Highly viscous sodium hyaluronate and joint lubrication. Int Orthop 2004;26:116–121.
14. Allard S, O’Regan M. The role of elastoviscosity in the efficacy of viscosupplementation for osteoarthritis of the knee: a comparison of Hylan G-F 20 and a lower molecular weight hyaluronan. Clin Ther 2000;22:792–795.
15. Biomet Inc. Technical literature: Fermathron™. fr.biomet.be/befr-medical/befr-biomaterials/befr-fermathron (accessed 17 September 2009).
16. Lindberg M, Lindberg P. Overcoming obstacles for adherence to phosphate binding medication in dialysis patients: a qualitative study. Pharm World Sci 2008;30:571–576.
17. Jani R et al. Ion exchange resins for ophthalmic delivery. J Ocul Pharmacol 1994;10:57–67.
18. Higuchi T. Mechanism of sustained-action medication. Theoretical analysis of rate of release of solid drugs dispersed in solid matrices. J Pharm Sci 1963;52:1145–1149.
19. Santus G, Baker RW. Osmotic drug delivery: a review of the patent literature. J Control Release 1995;35:1–21.
20. Gupta BP et al. Osmotically controlled drug delivery systems with associated drugs. J Pharm Pharm Sci 2010;13:571–588.
21. Theeuwes F et al. Osmotic delivery systems for the alpha-adrenoceptor antagonists metoprolol and oxyprenolol: design and evaluation of systems for once-daily administration. Br J Clin Pharmacol 1985;19(Suppl):69S–76S.
22. Wolff M et al. In vitro and in vivo release of nitroglycerin from a new transdermal therapeutic system. Pharm Res 1985;2:23–29.
23. Abdelrahman T, Wilson H. Wound dressings: principles and practice. Surgery 2011;29:491–495.
24. Turner TD. Synthaderm – an ‘environmental’ dressing. Pharm J 1982;228:206–208.
25. Myers JA. Lyofoam – a versatile polyurethane foam surgical dressing. Pharm J 1985;235:270.
Further reading
Chakraborty S et al. Electrohydrodynamics: a facile technique to fabricate drug delivery systems. Adv Drug Del Rev 2009;61:1043–1105.
Liechty WB et al. Polymers for drug delivery. Annu Rev Chem Biomol Eng 2010;1:149–173.
Mogoşanu GD, Grumezescu AM. Natural and synthetic polymers for wounds and burns dressings. Int J Pharm 2014;463:127–136.
Siepmann F et al. Polymer blends for controlled release coatings. J Control Rel 2008;125:1–15.
Tong L et al. Choice of artificial tear formulations for patients with dry eye. Cornea 2012;31(Suppl. 1): S32–S36.
Zaman M et al. Advances in drug delivery via electrospun and electrosprayed materials. Int J Nanomed 2013;8:2997–3017.
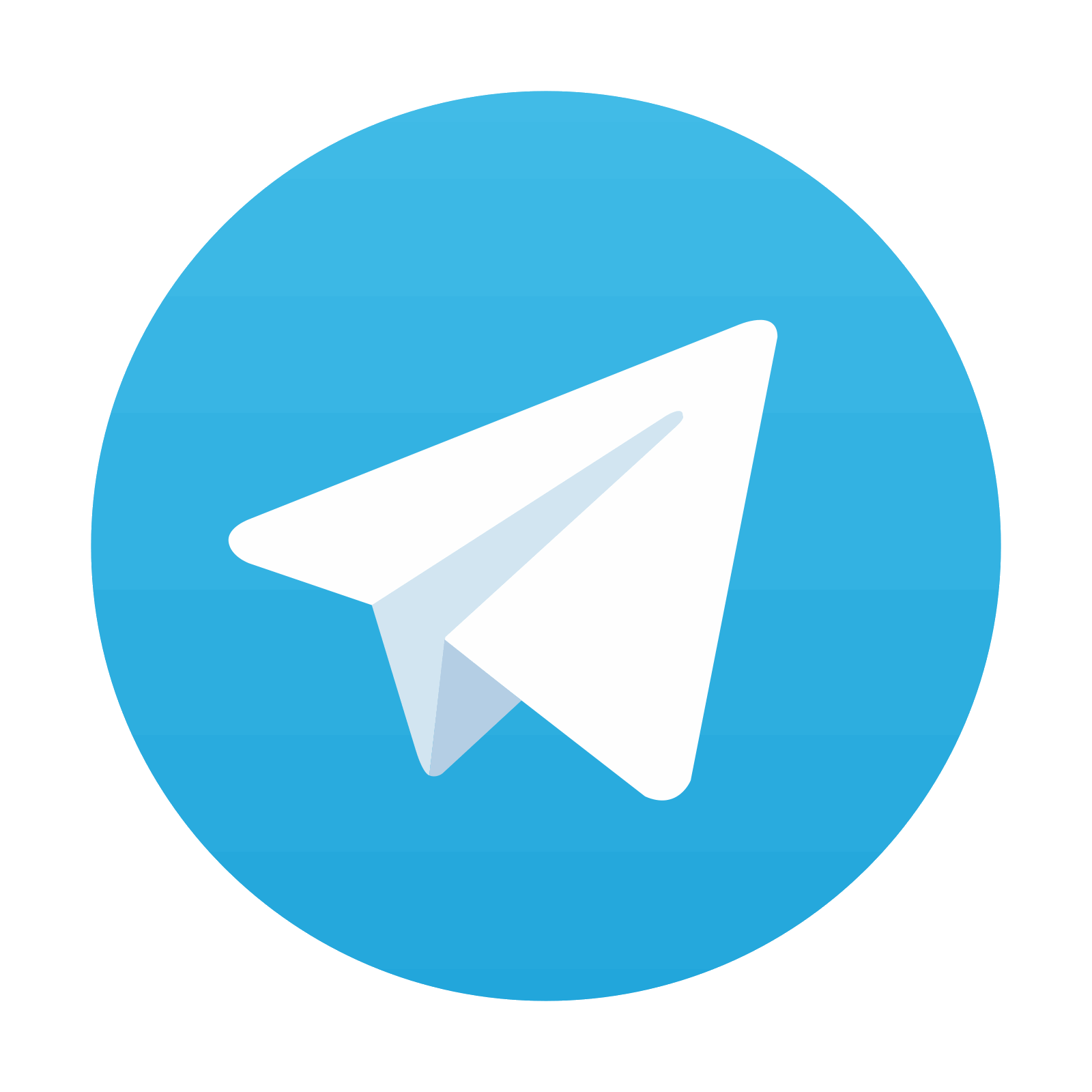
Stay updated, free articles. Join our Telegram channel
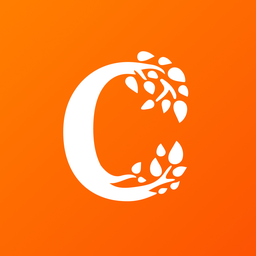
Full access? Get Clinical Tree
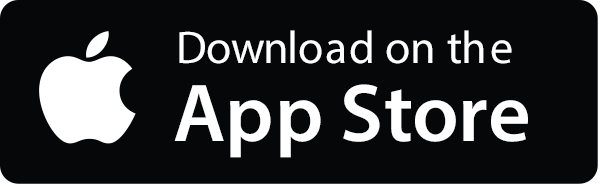
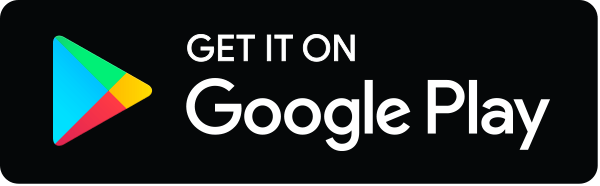