Anthony Hollenberg and William W. Chin
The thyroid gland has diverse and important effects on many aspects of metabolic homeostasis. Follicular thyroid cells constitute the majority of thyroid tissue; these cells produce and secrete the classical thyroid hormones: thyroxine (T4), triiodothyronine (T3), and reverse triiodothyronine (rT3). Thyroid hormones regulate growth, metabolism, and energy expenditure, from oxygen consumption to cardiac contractility. Parafollicular C cells of the thyroid gland secrete calcitonin, a regulator of bone mineral homeostasis. Calcitonin is discussed in Chapter 32, Pharmacology of Bone Mineral Homeostasis.
The major diseases of the thyroid gland involve disruption of the normal hypothalamic-pituitary-thyroid axis (see Chapter 27, Pharmacology of the Hypothalamus and Pituitary Gland). Replacement of deficient thyroid hormone is an effective and established therapy for hypothyroidism. Treatment of hyperthyroidism is more complex, with options including antithyroid drugs, radioactive iodine, and surgical excision of abnormal tissue. Understanding the pathways and mechanisms of feedback regulation of thyroid hormone synthesis and thyroid hormone actions serves to explain the rationale for effective drug treatment of thyroid diseases.
Over the course of a few months, Diana L, 45 years old, notices a number of disconcerting changes in the way she feels and in her general appearance. Ms. L feels nervous all the time; small events make her jumpy. She also keeps the temperature unusually cold in her house, to the point where her husband and children begin to complain. Because of these symptoms and the occasional feeling that her heart “skips a beat,” Ms. L goes to see her doctor. After some questioning, he palpates her neck and notes that her thyroid gland is diffusely enlarged. He also notes that Ms. L’s eyes are more prominent than normal. Tests for thyroid hormone levels reveal high serum free triiodothyronine (T3) and low thyrotropin (TSH). In addition, a test for TSH receptor antibody is positive. Ms. L is diagnosed with Graves’ disease, a form of hyperthyroidism, and treated with methimazole. Although initially comforted by the fact that her doctor can explain her symptoms, she soon becomes discouraged because she does not notice any improvement for a couple of weeks. After a month, however, her symptoms begin to subside. Repeat tests confirm that her thyroid hormone levels are normalized. One year after starting treatment with methimazole, however, she begins to reexperience palpitations and feels anxious. Her doctor confirms that her thyroid hormone levels are again elevated, despite methimazole therapy. After discussion with her doctor, Ms. L elects to undergo treatment with radioactive iodine. She tolerates the treatment well, and testing over the next 3 years shows that she has normal thyroid hormone levels. However, 4 years after radioactive iodine treatment, she develops symptoms that are the opposite of her original problems: she feels tired and cold all the time, and she gains 30 pounds over the course of 6 months. Her doctor confirms that Ms. L has developed hypothyroidism. He prescribes thyroxine (T4), which she now takes once a day, and she feels well again.
Questions
1. Why was Ms. L’s serum thyrotropin level low but her triiodothyronine level high?
2. Which features of the thyroid gland make radioactive iodine a generally safe and specific therapy for hyperthyroidism?
3. Why did Ms. L develop hypothyroidism after treatment with radioactive iodine?
4. What is the mechanism of action of methimazole? Why did methimazole eventually stop working?
Synthesis and Secretion of Thyroid Hormones
The thyroid is an endocrine gland located in the neck inferior to the larynx and spanning the ventral surface of the trachea. The main function of the thyroid gland is to produce the thyroid hormones, T3 and T4. Structurally, the thyroid hormones are built on a backbone of two tyrosine molecules that are iodinated and connected by an ether linkage (Fig. 28-1). An important structural feature of thyroid hormones is the placement of iodines on this backbone. The position and relative orientation of iodines attached to the tyrosine residues determine the specific form of thyroid hormone. 3,5,3′,5′-Tetraiodothyronine (thyroxine, T4) has four iodines attached to the tyrosine backbones and is the major form of thyroid hormone secreted by the thyroid gland. 3,5,3′-Triiodothyronine (T3) has three iodines. Most T3 is produced by peripheral or extra-thyroidal 5′ deiodination of T4 (see below). A biologically inactive form of thyroid hormone is 3,3′,5′-triiodothyronine, also referred to as reverse triiodothyronine (rT3) because the single iodine is on the opposite tyrosine in the backbone relative to T3. In a normal individual, circulating thyroid hormone consists of about 90% T4, 9% T3, and 1% rT3, and most of the hormone is bound to plasma proteins (both specific binding proteins and albumin).
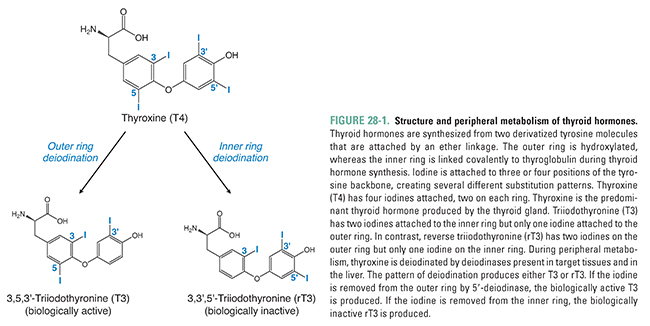
Iodine is a trace element that is a crucial component of thyroid hormone structure. Thyroid follicular cells, which synthesize and secrete thyroid hormones, selectively concentrate iodide (I−) via a Na+/I− symporter (NIS) located on the basolateral membrane of the cell (Fig. 28-2). This active transport mechanism has the ability to concentrate iodide to intracellular concentrations up to 500 times that of plasma; most individuals have thyroid gland to plasma iodide ratios of approximately 30.
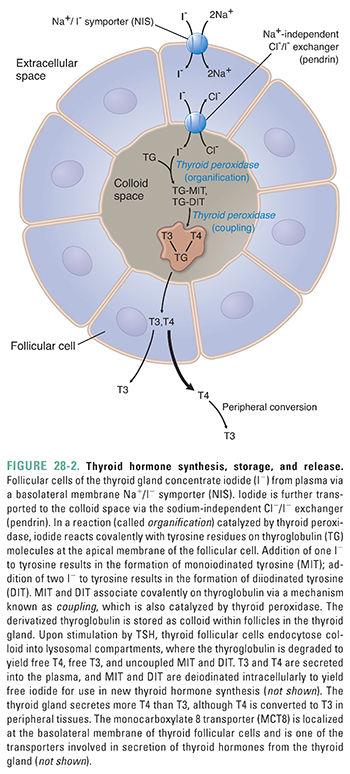
Once inside thyroid gland follicular cells, iodide is transported across the apical membrane of the cell via a Na+-independent Cl−/I− exchanger (one is known as pendrin) into the colloid space (Fig. 28-2). Iodide is then oxidized by the enzyme thyroid peroxidase (Fig. 28-2). This oxidation reaction creates a reactive iodide intermediate that couples to specific tyrosine residues on thyroglobulin. Thyroglobulin is a protein synthesized by thyroid follicular cells and secreted at the apical surface into the colloid space. Thyroid peroxidase is also concentrated at the apical surface, and it is thought that generation of oxidized iodide at this surface allows the iodide to react with tyrosine residues in the newly secreted thyroglobulin molecules. The process of thyroglobulin iodination is known as organification. Organification results in thyroglobulin molecules containing monoiodotyrosine (MIT) and diiodotyrosine (DIT) residues; these tyrosine residues have one or two covalently attached iodines, respectively.
After MITs and DITs are generated within thyroglobulin, thyroid peroxidase also catalyzes coupling between these residues. An MIT joined to DIT generates T3, while the joining of two DITs creates T4. Note again that the majority of plasma T3 is produced by metabolism of T4 in the circulation (also see the following section, “Metabolism of Thyroid Hormones”) and that nascent T3 and T4 are covalently part of the thyroglobulin protein at this point. These thyroglobulin molecules are then stored in the lumen of the follicle as colloid.
When thyroid-stimulating hormone (discussed below) stimulates the thyroid gland to secrete thyroid hormone, the follicular cells endocytose colloid. The ingested thyroglobulin enters lysosomes, where proteases digest the thyroglobulin. Proteolytic digestion releases free T3, T4, MIT, and DIT. T3 and T4 are transported across the follicular cell basolateral membrane and into the blood. Free MIT and DIT are rapidly deiodinated within the cell, allowing the iodide to be recycled for new thyroid hormone synthesis.
Most endocrine organs concurrently synthesize and release new hormone when activated rather than storing large quantities of precursor hormone. The thyroid gland is unusual among endocrine glands in that it stores large quantities of thyroid prohormone in the form of thyroglobulin. It is not understood why the thyroid gland maintains this elaborate pathway for hormone synthesis and release, but doing so makes it possible to maintain plasma thyroid hormone at a constant level despite fluctuations in the availability of dietary iodide.
Metabolism of Thyroid Hormones
Thyroid hormone circulates mostly bound to plasma proteins, notably thyroid-binding globulin (TBG) and transthyretin. Although T4 is the predominant thyroid hormone found in the blood, the physiologic activity of T3 is fourfold higher than that of T4 on target tissues. Some serum T4 is inactivated by deamination, decarboxylation, or conjugation and excretion by the liver. Most T4, however, is deiodinated to the more active T3 form in several locations in the body. This deiodination reaction is catalyzed by the enzyme iodothyronine 5′-deiodinase (Fig. 28-1).
There are three subtypes of deiodinase. Type I 5′-deiodinase, expressed in the liver and kidneys, is important for converting T4 to the majority of serum T3. Type II 5′-deiodinase is expressed primarily in the pituitary gland, brain, and brown fat. This enzyme is located intracellularly and converts T4 to T3 locally. Type III 5-deiodinase is responsible largely for conversion of T4 to the biologically inactive rT3.
The presence of T4 in the blood provides a buffer, or reservoir, for thyroid hormone effects. Most T4 to T3 conversion occurs in the liver, and many pharmacologic agents that increase hepatic cytochrome P450 enzyme activity also increase T4 to T3 conversion. In addition, T4 has a half-life in the plasma of approximately 6 days, whereas plasma T3 has a half-life of only 1 day. Because T4 has a long plasma half-life, changes in thyroid hormone-regulated functions caused by pharmacologic intervention are generally not observed for a period of 1 to 2 weeks, as seen with Ms. L in the introductory case.
Effects of Thyroid Hormones on Target Tissues
Thyroid hormones have effects on virtually every cell of the body. While the majority of the effects of thyroid hormones likely occur at the level of gene transcription, there is growing evidence that these hormones also act at the plasma membrane and/or in the cytoplasm. Both modes of action are mediated by hormone binding to thyroid hormone receptors (TRs).
Free hormone enters the cell by both passive diffusion and active transport, the latter mediated by hormone-specific and nonspecific carriers such as organic anion and monocarboxylate transporters that are located in the plasma membrane. The best described of these is the monocarboxylate 8 transporter (MCT8), although other transporters likely also contribute to thyroid hormone transport. Once inside the cell, thyroid hormone binds to thyroid hormone receptors. These TRs are proteins containing thyroid hormone-binding, DNA-binding, and dimerization domains. There are two classes of thyroid hormone receptor, termed TRα and TRβ. In addition, both TRα and TRβ can be expressed as multiple isoforms. TR monomers can interact in a dimerization reaction to form homodimers or with another transcription factor, retinoid X receptor (RXR), to form heterodimers. These TR dimers bind to gene regulatory regions and are activated by binding of thyroid hormones. Together, the multiple different combinations of TRs and the variability in their tissue distributions create tissue specificity for thyroid hormone effects.
In the absence of hormone, thyroid hormone receptor dimers associate with corepressor molecules and constitutively bind to (and thereby inactivate) thyroid hormone-stimulated genes. Binding of thyroid hormone to TR:RXR or TR:TR dimers promotes dissociation of the corepressors and recruitment of coactivators to the DNA. Thus, thyroid hormone binding to TR dimers serves as a molecular switch from inhibition to activation of gene transcription (Fig. 28-3). Thyroid hormone also acts to down-regulate gene expression by a TR-dependent mechanism, the exact nature of which is not fully understood. For example, thyroid hormone is able to down-regulate TSH subunit gene expression, causing negative feedback of thyroid hormone on the hypothalamic-pituitary-thyroid axis (see Chapter 27). Increasing evidence suggests that thyroid hormone also has nongenomic effects on mitochondrial metabolism and that it interacts with plasma membrane receptors to stimulate intracellular signal transduction.
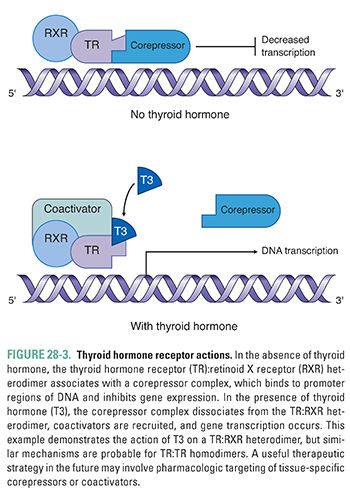
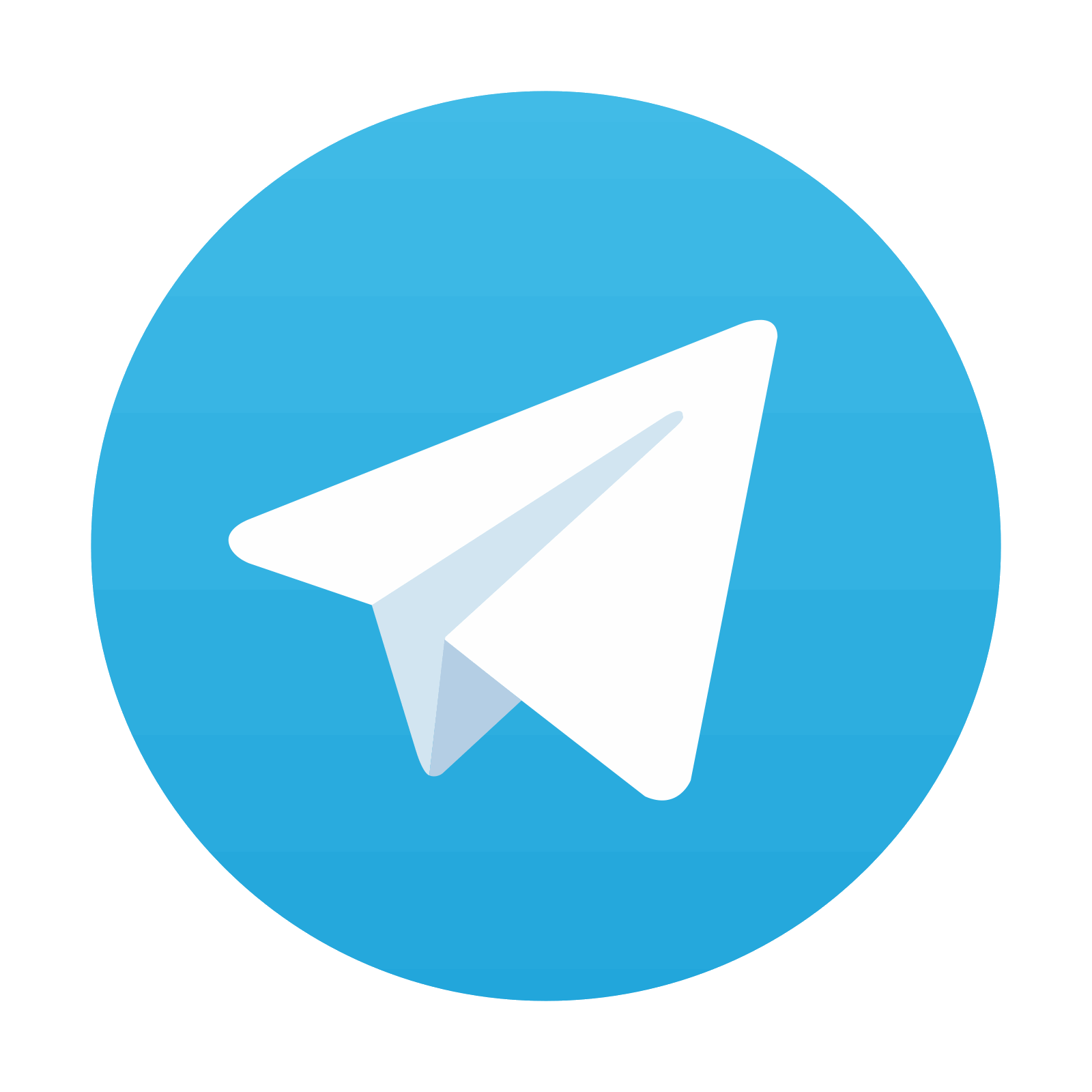
Stay updated, free articles. Join our Telegram channel
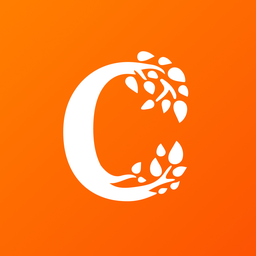
Full access? Get Clinical Tree
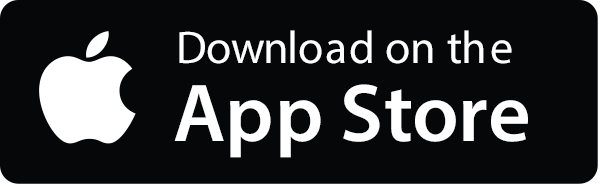
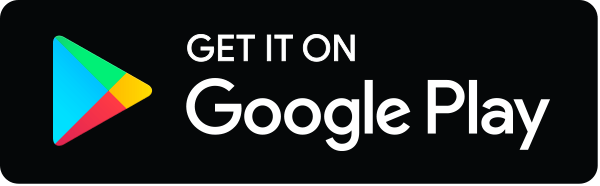