Andrew J. Wagner, Ramy A. Arnaout, and George D. Demetri
Recombinant Human G-CSFs (Filgrastim and PEG-Filgrastim) and GM-CSF (Sargramostim) | |
Many clinical situations are characterized by deficiencies of red blood cells, white blood cells, and/or platelets—cells of the hematopoietic system. This chapter describes the pharmacologic agents that can be used to stimulate production of hematopoietic cells; it is also important to note the nonpharmacologic alternatives, which could include transfusion and bone marrow transplantation. Blood cell production is controlled physiologically by hematopoietic growth factors, a diverse but functionally overlapping group of glycoproteins produced by the body in response to certain signals. For example, hypoxia stimulates the synthesis and release of the erythroid lineage growth factor erythropoietin, which in turn stimulates the production of erythrocytes in an attempt to relieve the hypoxia. The main pharmacologic strategy used to stimulate the production of blood cells is to administer exogenous growth factors or synthetic growth factor analogues. This chapter provides an introduction to the cells of the hematopoietic system, the growth factors that stimulate their production, and the pharmacologic agents used to increase blood cell production. An outline of the immunomodulatory agents used in anticancer therapy is also presented.
Fifty-two-year-old Mrs. M presents with a lump in her left breast. Subsequent mammogram, core biopsy, and lumpectomy lead to the diagnosis of infiltrating ductal carcinoma that is localized but lymph node–positive. She begins adjuvant chemotherapy with doxorubicin and cyclophosphamide. Ten days after the first cycle of chemotherapy, her white blood cell count (WBC) drops, as expected; over the next 9 days, her WBC recovers to its normal value. By the third cycle of chemotherapy, Mrs. M is moderately anemic, with a hematocrit of 28% (normal, 37–48%), and she feels quite fatigued. Seven days after the fourth cycle of chemotherapy, her WBC plummets to 800 cells per microliter (μL) of blood (normal, 4,300–10,800 cells/μL), and her absolute neutrophil count (ANC) is 300 cells/μL. In this setting, she develops shaking chills and a fever to 102°F. She is admitted to the hospital, where she receives parenteral antibiotics, and she remains there for 5 days until her ANC rises to an acceptable level. Mrs. M completes her cycles of doxorubicin and cyclophosphamide chemotherapy, continues chemotherapy with paclitaxel, and receives local radiation therapy.
Mrs. M is well for 2 years but then presents with pain in the left leg. Workup reveals that the cancer has metastasized to her left femur and liver. She begins chemotherapy with doxorubicin and docetaxel but again develops severe neutropenia and fever. She becomes short of breath while climbing stairs, and her hematocrit is 27%. Her iron stores are normal. Thereafter, her chemotherapy is supplemented with pegylated recombinant human G-CSF (PEG-filgrastim) and an analogue of human erythropoietin (darbepoetin). Neutropenia and fever do not recur, and by 4 weeks after the initiation of erythropoietin therapy, her hematocrit rises to 34.5% and she has normal exercise tolerance. The chemotherapy yields excellent palliative results. One year later, she is still in remission and leading an active life.
Questions
1. Are G-CSF and erythropoietin multilineage growth factors or lineage-specific growth factors?
2. How does erythropoietin increase the number of erythrocytes in the blood?
3. How do analogues of hematopoietic growth factors such as darbepoetin and PEG-filgrastim differ from endogenous, “natural” hematopoietic growth factors?
4. What are the important adverse effects of erythropoietin?
The cells of the hematopoietic system are functionally diverse (Table 45-1). Red blood cells, or erythrocytes, carry oxygen; many types of white blood cells, from granulocytes and macrophages to lymphocytes, fight infection and help protect against cancer; and platelets help control bleeding. Nonetheless, these cells have one feature in common: they all develop from a common cell in the bone marrow called the pluripotent hematopoietic stem cell (Fig. 45-1). Hematopoietic stem cells are induced to differentiate along committed lineages into red blood cells, white blood cells, or platelets through interactions with glycoproteins called hematopoietic growth factors.
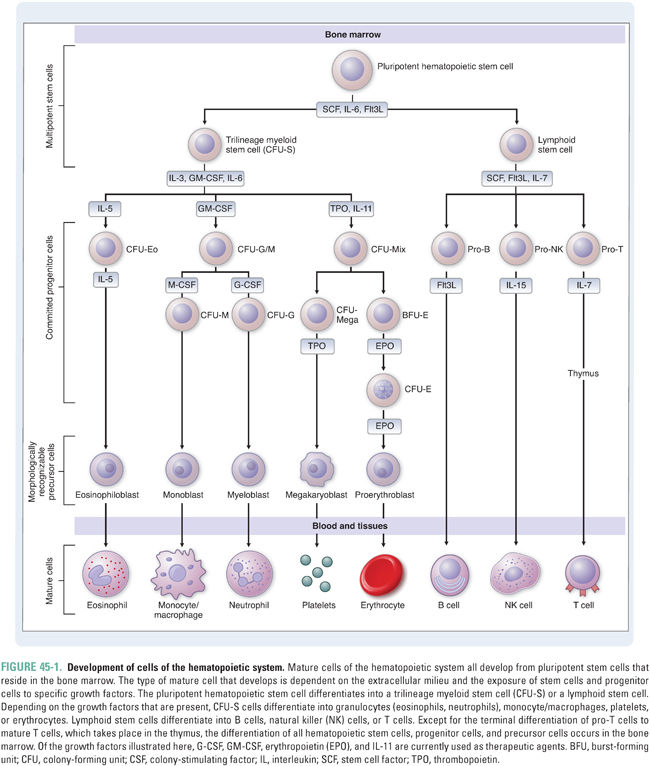
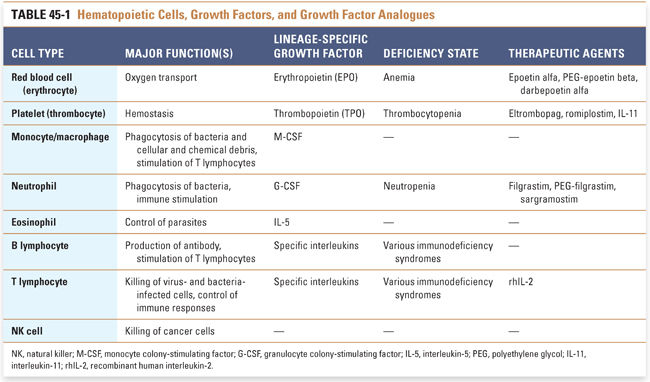
Central Role of Hematopoietic Growth Factors
Hematopoietic growth factors and cytokines constitute a heterogeneous group of molecules that regulate blood cell production, maturation, and function. Nearly 36 such factors have been identified, ranging in size from 9 to 90 kDa. The membrane-associated receptors for these factors belong to at least six receptor superfamilies, and genes encoding the factors are found on 11 different chromosomes. Conceptually, growth factors can be divided into two groups: multilineage (also called general, early-acting, or pleiotropic) growth factors, which stimulate multiple lineages, and lineage-specific (also called lineage-dominant or late-acting) growth factors, which stimulate differentiation and survival of a single lineage. Many growth factors and cytokines act synergistically with one another, sometimes with overlapping effects.
Multilineage growth factors include stem cell factor (also called steel factor or KIT ligand), interleukin-3 (IL-3), granulocyte-monocyte colony-stimulating factor (GM-CSF), insulin-like growth factor 1, IL-9, IL-11, and others. Many of these growth factors are discussed below with respect to the development of individual hematopoietic cell types. The relevant pharmacologic principle is that multilineage growth factors might be appropriate for treating conditions such as pancytopenia in which multiple hematopoietic lineages are affected.
The ability of multilineage growth factors to stimulate multiple lineages results from two features of their molecular and cellular physiology. First, the receptors for these growth factors are both structurally related and modular; this commonality makes them somewhat interchangeable. Second, the signal transduction cascades activated by binding of these growth factors to their receptors involve a common family of signaling proteins, the JAK-STAT proteins. In the myeloproliferative diseases polycythemia vera, essential thrombocytosis, and myeloid metaplasia with myelofibrosis, the JAK2 kinase is constitutively activated by a point mutation that leads to a single amino acid substitution (V617F) in the protein product of the gene. These diseases are characterized by clonal proliferation of all lineages, highlighting the general role of the JAK-STAT pathway in hematopoiesis. Pharmacologists have exploited the commonalities of multilineage growth factor signaling to design synthetic growth factors with novel properties (see below).
Lineage-Specific Growth Factors
For a growth factor to be lineage-specific, at least one of two conditions must be met: (1) the expression of the growth factor’s receptor(s) must be limited to progenitor and/or precursor cells within a single lineage and/or (2) the growth factor must induce inhibitory or apoptotic signals in cells of other lineages. Erythropoietin is one example of a lineage-specific growth factor; thrombopoietin, whose actions are essentially limited to the platelet lineage, is another. Other so-called lineage-specific growth factors are more properly considered lineage-selective, because they have secondary effects on lineage(s) other than the lineage of their primary action. Such factors include G-CSF, which primarily promotes the differentiation of neutrophils, and a number of interleukins, which have selective actions on certain myeloid and lymphoid lineages (see below). From a pharmacologic perspective, lineage-specific growth factors represent selective therapeutics that can be used to treat a deficiency of a single hematopoietic cell type. Some growth factors may also have unique effects against certain cancers, perhaps due to their prodifferentiation and promaturation properties.
Erythrocyte Production (Erythropoiesis)
Erythrocytes are uniquely suited to their role of transporting oxygen from the lungs to the tissues of the body. These cells contain high concentrations of hemoglobin, a protein that binds and releases oxygen molecules in response to the partial pressure of oxygen in the blood and tissues. Each hemoglobin molecule consists of four similar polypeptide chains, and each chain contains a binding site for molecular oxygen. The major form of adult hemoglobin, which has two alpha and two beta chains (α2β2), is called hemoglobin A (HbA). Fetal hemoglobin, or hemoglobin F (HbF), contains gamma (γ) chains instead of β chains (α2γ2). HbF predominates during the latter 6 months of fetal life and has a higher affinity for oxygen than HbA does, which helps facilitate the transfer of oxygen from mother to fetus. After birth, DNA methylation inactivates the γ globin gene, and expression of the β globin gene rises. It is important to note that expression of the α, β, and γ globin chains is regulated independently, making possible a multitude of hemoglobinopathies in which the α or β chains are abnormal or underexpressed because of an inherited mutation. In sickle cell anemia, a point mutation in the β globin gene results in the production of an abnormal hemoglobin—hemoglobin S (HbS)—that polymerizes upon deoxygenation, causing morphologic “sickling” of erythrocytes and leading to hemolytic anemia, painful vaso-occlusive crises, and profound end-organ damage. This autosomal recessive disease is the most common inherited blood disorder in the United States, affecting more than 70,000 individuals. Another common hemoglobinopathy is β thalassemia, in which the β chain is structurally and functionally normal but underexpressed.
Upon their release from the bone marrow, normal erythrocytes circulate in the blood with a lifespan of approximately 120 days. The number of erythrocytes in the blood is determined by the balance between new erythrocyte production in the bone marrow and erythrocyte loss due to cell destruction (hemolysis) and bleeding. This number is measured clinically as either the hemoglobin level (the concentration of hemoglobin per unit volume of blood) or the hematocrit (the percentage of blood volume that is composed of erythrocytes). The normal hemoglobin level ranges from 14 g/dL to 17 g/dL in men and 12 g/dL to 15 g/dL in women, and the normal hematocrit ranges from 42% to 50% in men and 37% to 46% in women. These gender differences are often attributed to increased blood loss through physiologic—that is, menstrual—bleeding in women and enhanced erythropoiesis induced by androgens (through unclear mechanisms) in men. A hemoglobin level or hematocrit below the normal range is defined as anemia.
Erythrocyte production, or erythropoiesis, proceeds under the control of several growth factors. The major growth factor controlling erythropoiesis is erythropoietin, a heavily glycosylated protein that is produced mainly by the liver in the fetus and by the kidney after birth. A lineage-specific growth factor, erythropoietin has received great clinical attention because it stimulates all but the earliest intermediates in the erythroid lineage but does not significantly affect other lineages. Its physiologic importance is attested to by experiments in mice and pathologic conditions in humans, both of which show that the absence of erythropoietin results in severe anemia. Furthermore, rare activating mutations of the erythropoietin receptor have been described in patients with primary familial and congenital polycythemia, a disorder manifested by isolated erythrocytosis and increased responsiveness to erythropoietin. Such was the case for Eero Mantyranta, a Finnish cross-country skier who won several gold medals in the 1964 Olympics but was accused of blood “doping” (receiving erythrocyte transfusions to artificially increase oxygen-carrying capacity) because of an abnormally high hematocrit. He was exonerated 30 years later when researchers identified an activating mutation of the erythropoietin receptor in samples from him and his family.
Given the role of erythrocytes in transporting oxygen, it is not surprising that erythropoietin production is triggered by hypoxia. Erythropoietin expression is strongly induced by hypoxia-inducible factor 1 alpha (HIF-1α), which binds to an enhancer element in the erythropoietin gene and activates gene transcription (Fig. 45-2). The level of HIF-1α within a cell is heavily influenced by the local oxygen tension. Under normal or high oxygen conditions, HIF-1α is hydroxylated by prolyl hydroxylase (PHD) via its Fe (II)-dependent dioxygenase activity. Prolyl hydroxylation of HIF-1α facilitates its binding to the von Hippel-Lindau (pVHL) E3 ubiquitin ligase complex, thus targeting HIF-1α for proteasomal degradation. Under hypoxic conditions, prolyl hydroxylation of HIF-1α does not occur, HIF-1α does not associate with pVHL, and HIF-1α instead translocates to the nucleus, where it enhances transcription of the hypoxia-inducible genes, including erythropoietin. In the rare autosomal recessive disease familial erythrocytosis 2 (also called Chuvash polycythemia, after the ethnic population of the mid-Volga River region in which it was first described), both germline copies of pVHL are mutated so as to prevent association with HIF-1α, reducing the degradation of HIF-1α and leading to elevated levels of erythropoietin and other target genes.
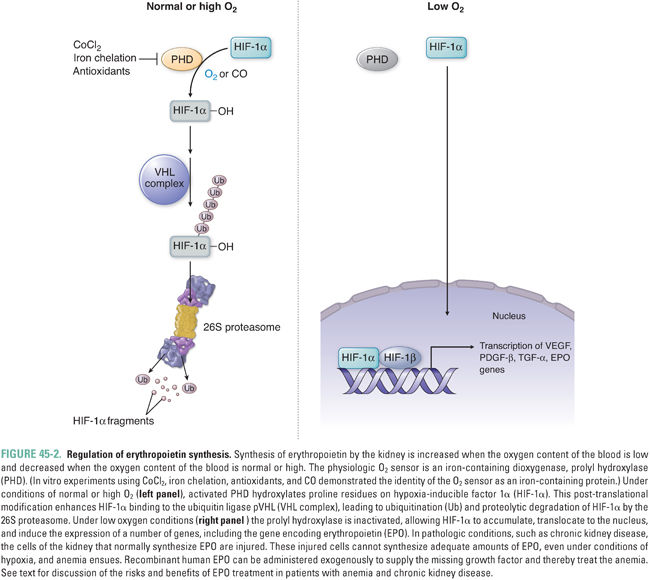
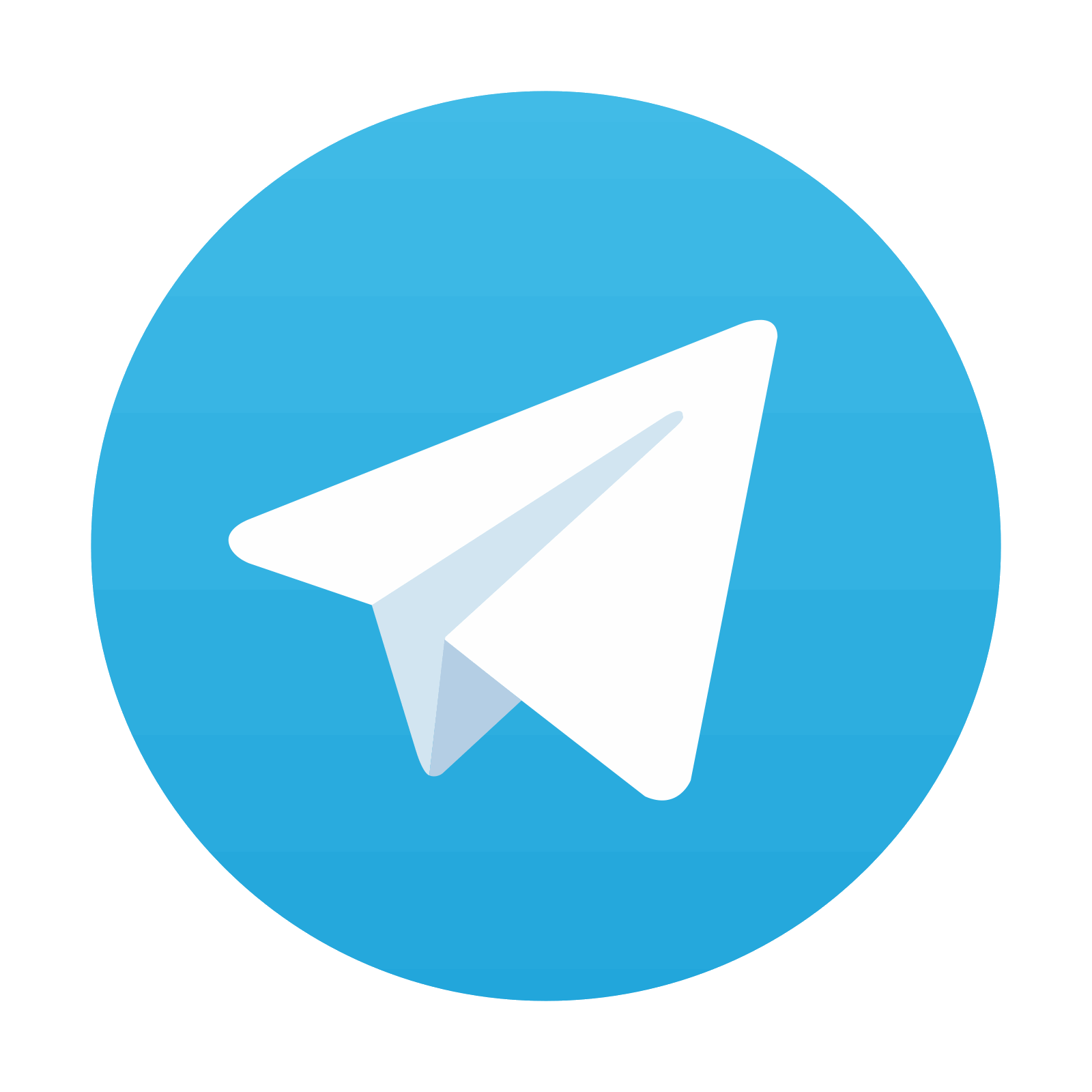
Stay updated, free articles. Join our Telegram channel
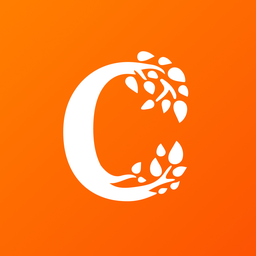
Full access? Get Clinical Tree
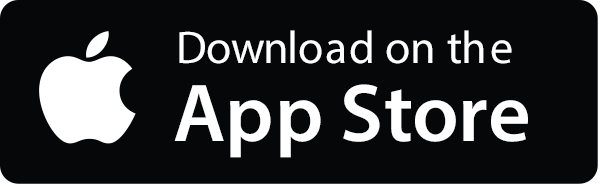
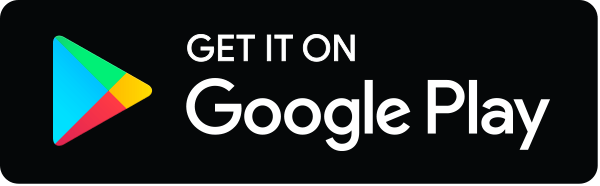