David G. Standaert and Victor W. Sung
BIOCHEMISTRY AND CELL BIOLOGY OF DOPAMINERGIC NEUROTRANSMISSION |
Dopamine (DA) is a catecholamine neurotransmitter that is the therapeutic target for a number of important central nervous system (CNS) disorders, including Parkinson’s disease and schizophrenia. DA is also a precursor for the other catecholamine neurotransmitters norepinephrine and epinephrine. The machinery of catecholamine neurotransmission has a number of components that are shared among members of the class, including biosynthetic and metabolic enzymes. There are also components that are specialized for the individual members of the class, including reuptake pumps and presynaptic and postsynaptic receptors. This chapter presents the principles that underlie current therapies for diseases that directly or indirectly involve changes in dopaminergic neurotransmission. The chapter begins with a discussion of the biochemistry and cell biology of dopaminergic neurotransmission and the localization of the major DA systems in the brain. Following this background, the chapter explores the physiology, pathophysiology, and pharmacology of Parkinson’s disease, which results from the specific loss of neurons in one of these DA systems, and schizophrenia, which is currently treated, in part, with drugs that inhibit dopaminergic neurotransmission.
Mark S is a 55-year-old man who goes to see his physician because he notices a tremor in his right hand that has developed gradually over a number of months. He finds he can keep the hand quiet if he concentrates on it, but the shaking quickly reappears if he is distracted. His handwriting has become small and difficult to read, and he has trouble using a computer mouse. His wife complains that he never smiles anymore and that his face is becoming expressionless. She also says that he walks more slowly and he has trouble keeping up with her. As Mr. S enters the examination room, his doctor notices that he is walking hunched over and has a short, shuffling gait. The doctor finds on physical examination that Mr. S has increased tone and cogwheel rigidity in his upper extremities, particularly on the right side, and that he is significantly slower than normal at performing rapid alternating movements. The physician determines that Mr. S’s symptoms and signs most likely represent the early stages of Parkinson’s disease, and she prescribes a trial of levodopa.
Questions
1. How does the selective loss of dopaminergic neurons result in symptoms such as those Mr. S is experiencing?
2. What will be the effect of levodopa on the course of Mr. S’s disease?
3. How will Mr. S’s response to levodopa change over time?
4. Is levodopa the best choice for Mr. S at this stage of his disease?
BIOCHEMISTRY AND CELL BIOLOGY OF DOPAMINERGIC NEUROTRANSMISSION
Dopamine belongs to the catecholamine family of neurotransmitters. In addition to dopamine, this family includes norepinephrine (NE) and epinephrine (EPI). As the name suggests, the basic structure of the catecholamines consists of a catechol (3,4-dihydroxybenzene) moiety connected to an amine group by an ethyl bridge (Fig. 14-1A). Recall from Chapter 9, Principles of Nervous System Physiology and Pharmacology, that catecholaminergic pathways in the brain have “single source-divergent” organization, in that they arise from small clusters of catecholamine neurons that give rise to widely divergent projections. CNS catecholamines modulate the function of point-to-point neurotransmission and affect complex processes such as mood, attentiveness, and emotion.
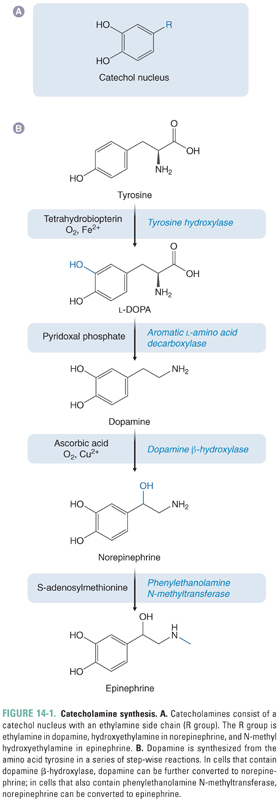
The neutral amino acid tyrosine is the precursor for all catecholamines (Fig. 14-1B). The majority of tyrosine is obtained from the diet; a small proportion may also be synthesized in the liver from phenylalanine. The first step in the synthesis of DA is the conversion of tyrosine to L-DOPA (L-3,4-dihydroxyphenylalanine, or levodopa) by oxidation of the 3 position on the benzene ring. This reaction is catalyzed by the enzyme tyrosine hydroxylase (TH), a ferro (iron containing)-enzyme that consists of four identical subunits of approximately 60 kDa each. In addition to Fe2+, TH also requires the cofactor tetrahydrobiopterin, which is oxidized to dihydrobiopterin in the course of the reaction. Importantly, oxidation of tyrosine to L-DOPA is the rate-limiting step in the production not only of DA but of all catecholamine neurotransmitters.
The next and final step in the synthesis of DA is the conversion of L-DOPA to DA by the enzyme aromatic L-amino acid decarboxylase (AADC). AADC cleaves the carboxyl group from the α-carbon of the ethylamine side chain, liberating carbon dioxide. AADC requires the cofactor pyridoxal phosphate. Although AADC is sometimes referred to as “DOPA decarboxylase,” it is promiscuous in its ability to cleave carboxyl groups from the α-carbons of all aromatic amino acids and is involved in the synthesis of noncatechol transmitters, such as serotonin. AADC is abundant in the brain. It is expressed by dopaminergic neurons, but it is also present in nondopaminergic cells and glia. Furthermore, AADC is expressed throughout the body in almost all cell types.
In dopaminergic neurons, the end product of the catecholamine synthetic pathway is dopamine. In cells that secrete the catecholamine NE, DA is converted to NE by the enzyme dopamine β-hydroxylase. In other cells, NE may subsequently be converted to epinephrine by phenylethanolamine N-methyltransferase. Dopaminergic neurons lack both of these enzymes, but it is important to keep in mind the entire pathway of catecholamine biosynthesis because pharmacologic manipulation of DA biosynthesis can also alter the production of NE and EPI. For a more complete discussion of the last two steps in NE and EPI synthesis, see Chapter 11, Adrenergic Pharmacology.
Dopamine Storage, Release, Reuptake, and Inactivation
DA is synthesized from tyrosine in the cytoplasm of the neuron and then transported into secretory vesicles for storage and release (Fig. 14-2). Two separate molecular pumps are required for the transport of DA into synaptic vesicles. A proton ATPase concentrates protons in the vesicle, creating an electrochemical gradient characterized by a low intravesicular pH (i.e., a high proton concentration) and an electropositive vesicle interior. This gradient is exploited by a proton antiporter, the vesicular monoamine transporter (VMAT), which allows protons to move down the gradient (out of the vesicle) while simultaneously transporting DA into the vesicle against its concentration gradient. Upon nerve cell stimulation, the DA storage vesicles fuse with the plasma membrane in a Ca2+-dependent manner, releasing DA into the synaptic cleft. In the cleft, DA can bind to both postsynaptic DA receptors and presynaptic DA autoreceptors (see below).
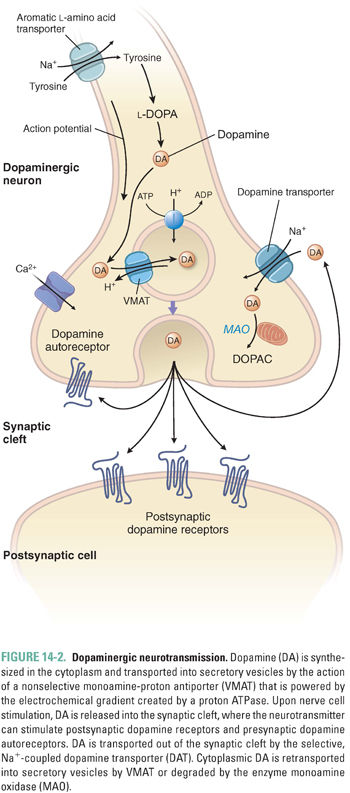
Several mechanisms exist to remove synaptic DA and terminate the signaling produced by the neurotransmitter. Most of the DA released into the synaptic cleft is transported back into the presynaptic cell by a 12-transmembrane domain protein, the dopamine transporter (DAT). DAT belongs to the family of catecholamine reuptake pumps. DA reuptake involves transport of the neurotransmitter against its concentration gradient and therefore requires an energy source. For this reason, the DAT couples dopamine reuptake to the co-transport of Na+ down its concentration gradient into the cell. In fact, both Na+ and Cl− are co-transported with DA into the cell. Because the Na+ gradient is maintained by the Na+/K+-ATPase pump, DA reuptake depends indirectly on the presence of a functioning Na+/K+ pump. DA taken up into the presynaptic cell can either be recycled into vesicles for further use in neurotransmission (by VMAT) or degraded by the action of the enzymes monoamine oxidase (MAO) or catechol-O-methyltransferase (COMT) (Fig. 14-3).
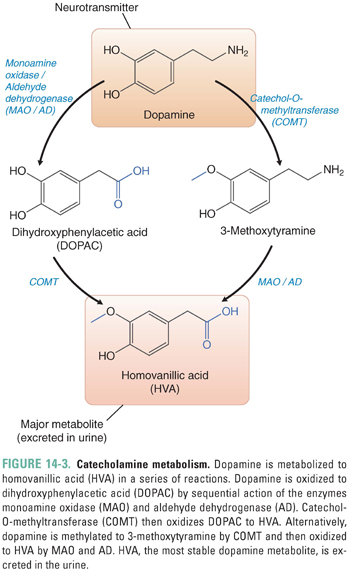
MAO is a key enzyme that functions to terminate the action of catecholamines in both the brain and the periphery. MAOs exist in two isoforms: MAO-A, which is expressed in the brain as well as the periphery, and MAO-B, which is concentrated in the CNS. Both isoforms of MAO can degrade dopamine as well as a wide range of monoamine compounds. Under normal conditions, MAO-B is responsible for catabolizing most CNS dopamine. The different roles played by the isoforms of MAO are therapeutically important. Selective inhibition of MAO-B is used to augment the function of CNS dopamine and generally is well tolerated. Inhibition of MAO-A, on the other hand, retards the breakdown of all central and peripheral catecholamines; as noted in Chapter 11, MAO-A inhibition may lead to life-threatening toxicity when combined with catecholamine releasers such as the indirect-acting sympathomimetic tyramine found in certain wines and cheeses.
Synaptic DA that is not taken up into the presynaptic cell can either diffuse out of the synaptic cleft or be degraded by the action of COMT. COMT is expressed in the brain, liver, kidney, and heart; it inactivates catecholamines by adding a methyl group to the hydroxyl group at the 3 position of the benzene ring. In the CNS, COMT is expressed primarily by neurons. The sequential action of COMT and MAO degrades DA to the stable metabolite homovanillic acid (HVA), which is excreted in the urine (Fig. 14-3).
Dopamine receptors are members of the G protein-coupled family of receptor proteins. The properties of dopamine receptors were originally classified by their effect on the formation of cyclic AMP (cAMP): activation of D1 class receptors leads to increased cAMP, while activation of D2 class receptors inhibits cAMP generation (Fig. 14-4). Subsequent studies led to cloning of the receptor proteins, revealing five distinct receptors, each encoded by a separate gene. All known DA receptors have the typical structure of G protein-coupled receptors, with seven-transmembrane domains. The D1 class contains two dopamine receptors (D1 and D5), while the D2 class contains three receptors (D2, D3, and D4). There are two alternative forms of the D2 protein, D2S (i.e., short) and D2L (i.e., long), which represent alternate splice variants of the same gene; their difference lies in the third cytoplasmic loop, which affects G protein interaction but not dopamine binding.
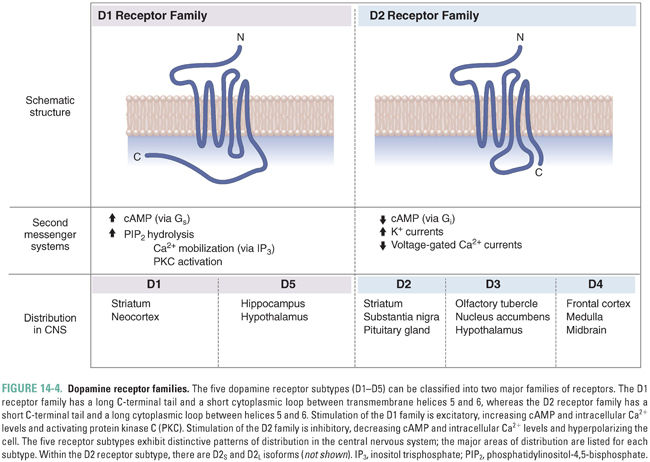
The five different dopamine receptor proteins have distinct distributions in the brain (Fig. 14-5). Both D1 and D2 receptors are expressed at high levels in the striatum (caudate and putamen), where they play a role in motor control by the basal ganglia, as well as in the nucleus accumbens (see Chapter 19, Pharmacology of Drugs of Abuse) and olfactory tubercle. D2 receptors are also expressed at high levels on anterior pituitary gland lactotrophs, where they regulate prolactin secretion (see Chapter 27, Pharmacology of the Hypothalamus and Pituitary Gland). D2 receptors are thought to play a role in schizophrenia because many antipsychotic medications have high affinity for these receptors (see below), although the localization of the D2 receptors involved remains to be elucidated. D3 and D4 receptors are structurally and functionally related to D2 receptors and may also be involved in the pathogenesis of schizophrenia. High levels of D3 receptors are expressed in the limbic system, including the nucleus accumbens and olfactory tubercle, while D4 receptors have been localized to the frontal cortex, diencephalon, and brainstem. D5 receptors are distributed sparsely and expressed at low levels, mainly in the hippocampus, olfactory tubercle, and hypothalamus.
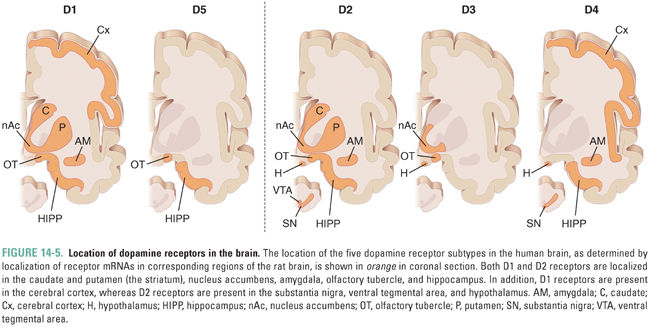
Regulation of cAMP formation is the defining characteristic of the dopamine receptor classes, but dopamine receptors can also affect other aspects of cellular function depending on their localization and linkage to second messenger systems. Most dopamine receptors are expressed on the surface of postsynaptic neurons at dopaminergic synapses. The density of these receptors is tightly controlled through regulated insertion and removal of dopamine receptor proteins from the postsynaptic membrane. DA receptors are also expressed presynaptically on the terminals of dopaminergic neurons. Presynaptic dopamine receptors, most of which are of the D2 class, serve as autoreceptors. These autoreceptors sense dopamine overflow from the synapse and reduce dopaminergic tone, both by decreasing DA synthesis in the presynaptic neuron and by reducing the rate of neuronal firing and dopamine release. Inhibition of DA synthesis occurs through cAMP-dependent down-regulation of TH activity, while the inhibitory effect on DA release and neuronal firing is due, in part, to a separate mechanism involving the modulation of K+ and Ca2+ channels. Increased K+ channel opening results in a larger current that hyperpolarizes the neuron, so that a larger depolarization is needed to reach the firing threshold. Decreased Ca2+ channel opening results in decreased levels of intracellular Ca2+. Because Ca2+ is required for synaptic vesicle trafficking to and fusion with the presynaptic membrane, decreases in intracellular Ca2+ levels result in decreased dopamine release.
Most central dopaminergic neurons originate in discrete areas of the brain, as shown in Figure 14-6 (see also Fig. 9-8), and have divergent projections. Three major pathways can be distinguished. The largest DA tract in the brain is the nigrostriatal system, which contains about 80% of the brain’s DA. This tract projects rostrally from cell bodies in the pars compacta of the substantia nigra to terminals that richly innervate the caudate and putamen, two nuclei that are collectively called the striatum. The striatum is named for the striped appearance of the white fiber tracts that run through it; the substantia nigra is named for the dark pigmentation that results from the decomposition of DA to melanin. Dopaminergic neurons of the nigrostriatal system are involved in the stimulation of purposeful movement. Their degeneration results in the abnormalities of movement that are characteristic of Parkinson’s disease.
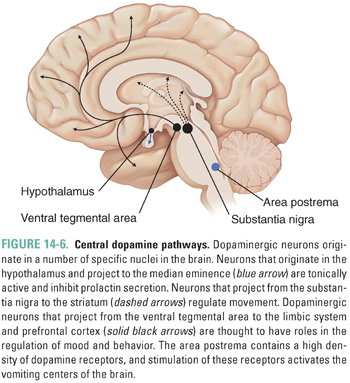
Medial to the substantia nigra is an area of dopaminergic cell bodies in the midbrain called the ventral tegmental area (VTA). The VTA has widely divergent projections that innervate many forebrain areas, most notably the cerebral cortex, the nucleus accumbens, and other limbic structures. These systems play an important and complex (as yet poorly understood) role in motivation, goal-directed thinking, regulation of affect, and positive reinforcement (reward). Derangement of these pathways may be involved in the development of schizophrenia; as discussed below, the blocking of dopaminergic neurotransmission can lead to a remission in psychotic symptoms. (See Chapter 19 for a more complete discussion of the reward pathway.)
DA-containing cell bodies in the arcuate and paraventricular nuclei of the hypothalamus project axons to the median eminence of the hypothalamus. This system is known as the tubero-infundibular pathway. Dopamine is released by these neurons into the portal circulation connecting the median eminence with the anterior pituitary gland and tonically inhibits the release of prolactin by pituitary lactotrophs.
A fourth anatomic structure, the area postrema located in the floor of the fourth ventricle, is also a target of dopaminergic therapies. The area postrema contains only a modest number of intrinsic dopamine neurons but a high density of dopamine receptors (mostly of the D2 class). The area postrema is one of the circumventricular organs that function as blood chemoreceptors. Unlike the rest of the brain, the blood vessels in the circumventricular organs are fenestrated, allowing communication between the blood and CNS (i.e., the circumventricular organs are “outside” the blood–brain barrier [BBB]). Stimulation of DA receptors in the area postrema activates the vomiting centers of the brain and is one of the causes of emesis. Drugs that block dopamine D2 receptors are used to treat nausea and vomiting.
A derangement in any of these dopaminergic systems can result in disease. Parkinson’s disease, which arises from dysregulated dopamine neurotransmission, and schizophrenia, which may also result from abnormal dopamine neurotransmission, are two such examples. These two diseases, and the pharmacologic interventions used to treat them, are highlighted below. Because the pharmacologic manipulation of dopaminergic systems is not always specific to one system, many of the adverse effects of drugs that act on these systems can be predicted based on their effects on the other dopaminergic systems.
DOPAMINE AND CONTROL OF MOVEMENT: PARKINSON’S DISEASE
Physiology of Nigrostriatal Pathways
The basal ganglia have a crucial role in the regulation of purposeful movement and are a site of the pathology in Parkinson’s disease. The basal ganglia do not connect directly to spinal motor neurons and thus do not directly control the individual movements of muscles. They appear to function instead by assisting in learning coordinated patterns of movement and by facilitating the execution of learned motor patterns. Dopamine has a central role in the operation of this system, including signaling when desired movements are executed successfully and driving the learning process.
Anatomically, the basal ganglia form a reentrant loop by receiving input from the cerebral cortex, processing this information in the context of dopaminergic input from the substantia nigra, and sending information back to the cortex by way of the thalamus. The internal circuitry of the basal ganglia consists of several components. The striatum (caudate and putamen) is the primary input nucleus of the system, while the globus pallidus pars interna and substantia nigra pars reticulata are the output nuclei. These are interconnected through two internuclei, the subthalamic nucleus and the globus pallidus pars externa.
Much of the information processing performed by the basal ganglia occurs in the striatum. The cortical inputs to this structure are excitatory and use glutamate as a transmitter. The striatum is also the target of the dopaminergic nigrostriatal pathway. The neurons in the striatum are of several types. The majority of neurons are “medium spiny” neurons. These cells are studded with spines that receive input from corticostriatal axons. These medium spiny neurons release the inhibitory transmitter GABA and send their projections to two downstream targets, forming the direct pathway and the indirect pathway (Fig. 14-7). The striatum also contains several small but important populations of interneurons, including neurons that release acetylcholine. These interneurons participate in the intercommunication between the direct and indirect pathways.
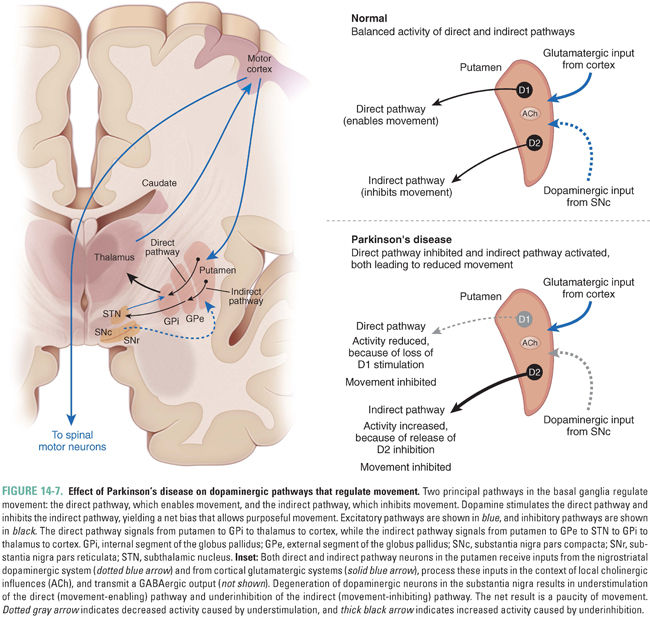
The balance of activity between the direct and indirect pathways regulates movement. The direct pathway, formed by striatal neurons expressing primarily dopamine D1 receptors, projects directly to the output of the basal ganglia, the internal segment of the globus pallidus. The latter neurons tonically inhibit the thalamus, which in turn, sends excitatory projections to the cortex that initiate movement. In this manner, activation of the direct pathway disinhibits the thalamus; that is, the activation of the direct pathway stimulates movement. The indirect pathway, formed by striatal neurons expressing predominantly D2 receptors, projects to the external segment of the globus pallidus, which in turn, inhibits neurons in the subthalamic nucleus. The neurons in the subthalamic nucleus are excitatory glutamatergic neurons that project to the internal segment of the globus pallidus. As a result of this multistep pathway, activation of the indirect pathway disinhibits neurons of the subthalamic nucleus, which, in turn, stimulate neurons in the internal segment of the globus pallidus to inhibit the thalamus; that is, the activation of the indirect pathway inhibits movement.
The differential expression of D1 and D2 receptors within the two pathways leads to differing effects of dopaminergic stimulation. Increased levels of dopamine in the striatum tend to activate the D1-expressing neurons of the direct pathway while inhibiting the D2-expressing neurons of the indirect pathway. Notice that both of these effects promote movement. The opposite effect occurs in Parkinson’s disease, a state of dopamine deficiency: the direct pathway shows reduced activity, while the indirect pathway is overactive, leading to reduced movement.
This model of basal ganglia function is greatly simplified, of course, but it has been useful in developing a deeper understanding of how the basal ganglia work. An important prediction of the model is that, in Parkinson’s disease, the indirect pathway (and, in particular, the subthalamic nucleus) should be overactive. This prediction has been proven directly by in vivo electrical recordings in patients with Parkinson’s disease. Furthermore, surgical therapies that target the subthalamic nucleus, such as deep brain stimulation in this location, are now often used to treat Parkinson’s disease when pharmacologic treatments are inadequate.
In Parkinson’s disease, there is a selective loss of dopaminergic neurons in the substantia nigra pars compacta (Fig. 14-7). The extent of loss is profound, with at least 70% of the neurons destroyed at the time symptoms first appear; often, 95% of the neurons are missing at autopsy. The destruction of these neurons results in the core motor features of the disease: bradykinesia, or slowness of movement; rigidity, a resistance to passive movement of the limbs; impaired postural balance, which predisposes to falling; and a characteristic tremor when the limbs are at rest.
The mechanisms underlying the destruction of DA neurons in the substantia nigra in Parkinson’s disease are not fully understood. Both environmental factors and genetic influences have been implicated. In 1983, the unexpected development of Parkinson’s disease in abusers of the synthetic opioid meperidine (see Chapter 18, Pharmacology of Analgesia) yielded the first agent known to produce Parkinson’s disease directly and the strongest evidence that environmental factors can cause Parkinson’s disease. These individuals, who tended to be young and otherwise healthy, suddenly developed severe, levodopa-responsive parkinsonian symptoms. The cases were all linked to a single contaminated batch of meperidine that had been synthesized in a makeshift lab. The contaminant was found to be 1-methyl-4-phenyl-1,2,3,6-tetrahydropyridine (MPTP), which forms as an impurity in the synthesis of meperidine when its manufacture is carried out for too long and at too high a temperature. Studies in nonhuman primates have shown that MPTP is oxidized in the brain to MPP+ (1-methyl-4-phenyl-pyridinium), which is selectively toxic to neurons in the substantia nigra. Despite extensive searches, it does not appear that there is any significant amount of MPTP present in the everyday environment, and MPTP itself is not the cause of most cases of Parkinson’s disease. There may, however, be other environmental factors that have a more subtle effect on development of the disease, such as exposure to certain pesticides.
Recent research has established that genetic factors contribute to Parkinson’s disease. The best-studied examples are families with mutations in or overexpression of the protein α-synuclein, which lead to autosomal dominant forms of Parkinson’s disease. While the function of this protein is not clear, it appears to be involved in the formation of neurotransmitter vesicles and the release of dopamine in the brain. At least four other genes have been identified as causing Parkinson’s disease in one or more families. These genetic discoveries have provided important clues into the biology of Parkinson’s disease and have allowed the development of transgenic mouse and fruit fly models that serve as a platform for developing new treatments. Although these genetic discoveries have provided insight into the biology of Parkinson’s disease, it is important to note that all of the different genetic causes identified so far account for less than 10% of cases, and most cases are still of unknown cause. The etiology of Parkinson’s disease in most patients is likely multifactorial, with contributions from both genetic and environmental factors.
Pharmacologic Classes and Agents
Parkinson’s disease is a progressive neurodegenerative disorder. Loss of dopaminergic neurons likely begins a decade or more before the symptoms become apparent, and this loss continues relentlessly. Currently available treatments are mostly symptomatic, meaning that they treat the symptoms but do not alter the underlying degenerative process. Symptomatic treatments are very useful and can restore function and quality of life for many years, but ultimately, the progression of the disease leads to increasing difficulty in managing the symptoms. In addition, some features of Parkinson’s disease do not respond well to current medications, particularly the nonmotor symptoms (such as cognitive impairment and dementia) that characterize the late stages of the disease and that result from an extension of the disease process from the dopaminergic system to other areas of the brain. The goal of much current research is the development of neuroprotective and neurorestorative therapies, which might delay or eliminate the need for symptomatic treatment and avoid the late complications of the disorder.
Most of the pharmacologic interventions currently used in Parkinson’s disease are aimed at restoring DA levels in the brain. In general, medications used in the management of Parkinson’s disease can be divided into DA precursors, DA receptor agonists, and inhibitors of DA degradation. There is a smaller but still useful role for the existing nondopaminergic therapies, such as anticholinergic agents that modify the function of striatal interneurons.
Levodopa was first used to treat Parkinson’s disease over 40 years ago and is still the most effective treatment for the disease. DA itself is not suitable because it cannot cross the BBB. However, DA’s immediate precursor, L-DOPA (levodopa), is readily transported across the BBB by the neutral amino acid transporter (see Chapter 9); once in the CNS, L-DOPA is converted to dopamine by the enzyme AADC. Thus, L-DOPA must compete with other neutral amino acids for transport across the BBB, and its availability in the CNS may be compromised by recent high-protein meals (see the introductory case in Chapter 9).
Orally administered levodopa is readily converted into dopamine by AADC in the gastrointestinal tract. This metabolic process both diminishes the amount of levodopa that can reach the blood–brain barrier for transport into the CNS and increases the peripheral adverse effects that result from the generation of dopamine in the peripheral circulation (predominantly nausea, due to binding of this dopamine to receptors in the area postrema). When levodopa is administered alone, only 1–3% of the administered dose reaches the CNS unchanged. In order to boost the levels of levodopa available to the brain and reduce the adverse effects of peripheral levodopa metabolism, levodopa is almost always administered in combination with carbidopa, an inhibitor of AADC (Fig. 14-8). Carbidopa effectively prevents the conversion of levodopa to DA in the periphery. Importantly, because carbidopa is not able to cross the BBB, it does not interfere with the conversion of levodopa to DA in the CNS. Carbidopa increases the fraction of orally administered levodopa available in the CNS from 1–3% (without carbidopa) to 10% (with carbidopa), allowing a significant reduction in the dose of levodopa and reducing the incidence of peripheral adverse effects.
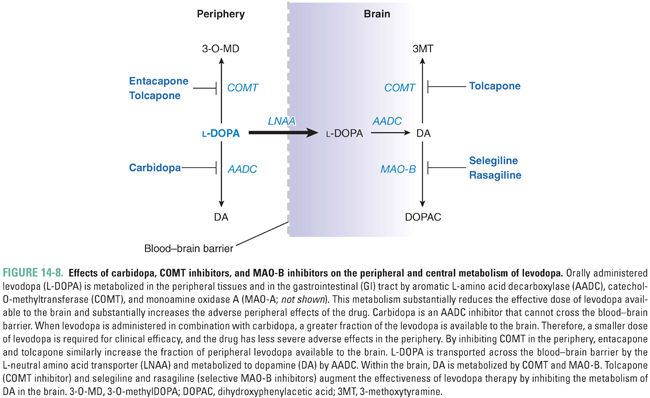
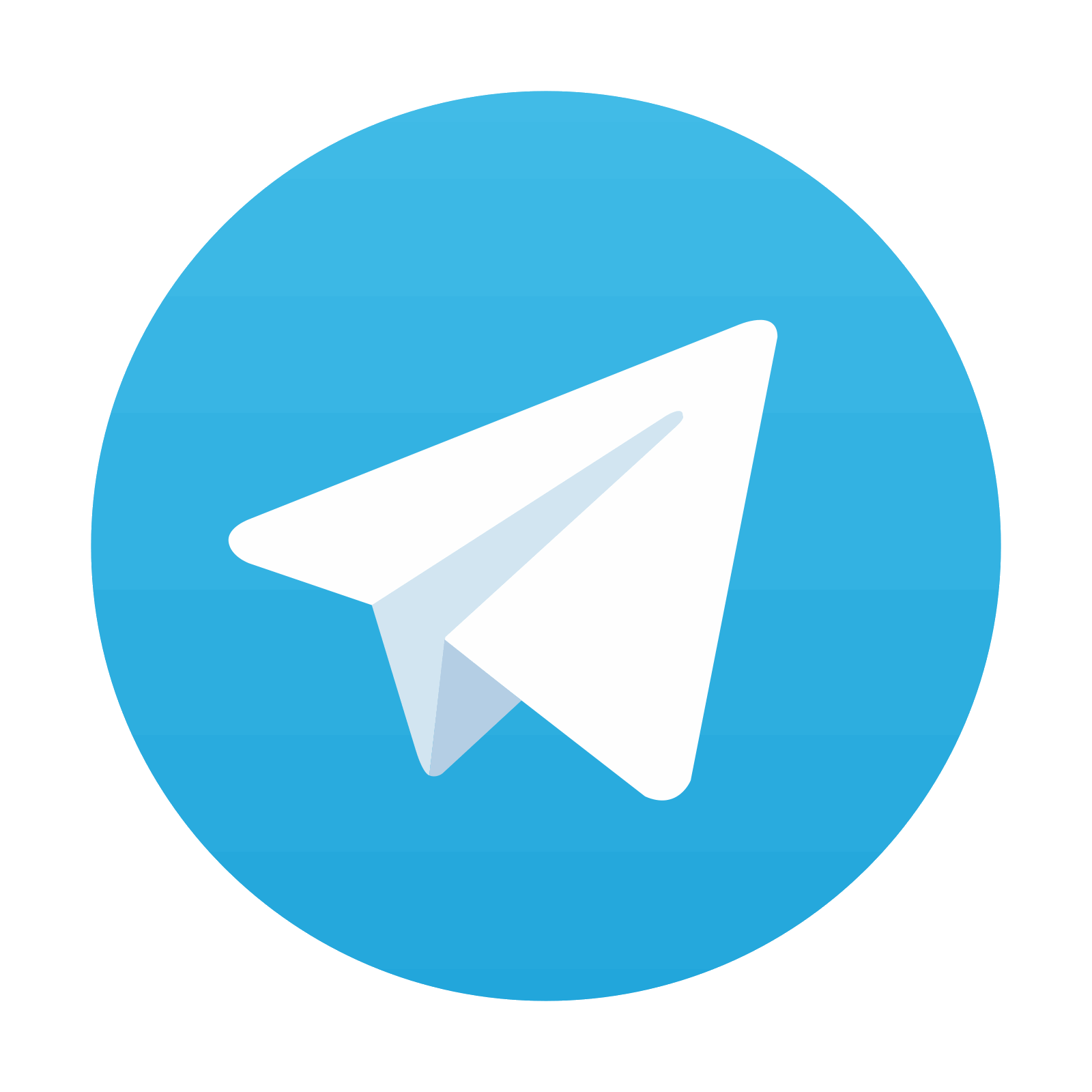
Stay updated, free articles. Join our Telegram channel
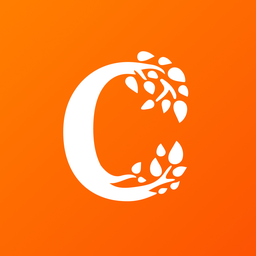
Full access? Get Clinical Tree
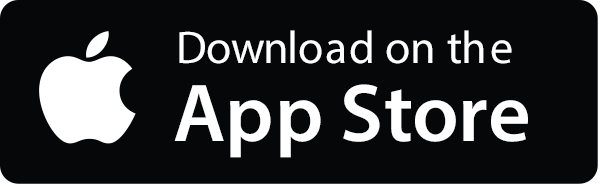
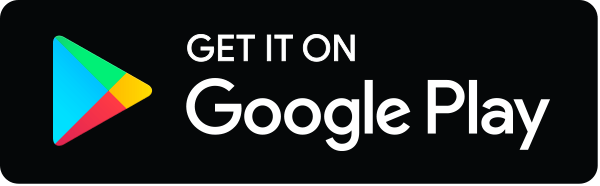