INTRODUCTION
In 1928, Alexander Fleming made a chance discovery that would revolutionize the treatment of bacterial infections. He observed that certain molds produce a compound that inhibits the growth of bacteria. The compound he isolated was penicillin, the first in a long line of antibiotics that act by inhibiting the biosynthesis of peptidoglycan, the major component of the bacterial cell wall. The unique chemical and structural properties of peptidoglycan make it an attractive and prominent target for antibacterial chemotherapy. The emergence and spread of antibiotic resistance increasingly complicate the clinical use of cell wall synthesis inhibitors, however. This chapter reviews the biochemistry of peptidoglycan synthesis and describes the mechanisms of action, uses, and limitations of the antibiotics that interfere with this pathway. These limitations include resistance, toxicity, and drug–drug interactions. Antibiotics that target other essential components of the bacterial cell wall are also discussed.
Samantha T is a 50-year-old woman who presents to an urgent care center with a sore area on her left medial thigh. She reports that she scratched her thigh on some exercise equipment at her local gym, where she works out 6 days a week. The area slowly became red, warm, and tender over the next 4 days. Over the past 24 hours, she has felt that the sore spot is “ready to burst.” On examination, Ms. T has a 2- × 2-cm, round, fluctuant area on the left thigh, with a surrounding patch of erythema that covers a 6- × 7-cm area of the thigh. She undergoes incision and drainage of the abscess in the urgent care office and the purulent material is sent for culture, which later grows methicillin-sensitive Staphylococcus aureus (MSSA). She is treated with dicloxacillin.
Ms. T returns to the urgent care clinic 10 days later and reports that the left thigh wound is nearly completely healed but that she now has profuse diarrhea and fever. She feels light-headed when standing. She reports that she has been living with her elderly father, who has several chronic medical ailments and was recently treated for Clostridium difficile infection. Ms. T is referred to her local emergency room for treatment of dehydration, and she is treated with intravenous fluids. A stool sample tests positive for Clostridium difficile toxin. Ms. T is treated with oral vancomycin and her diarrhea resolves over the course of the next week.
Questions
1. Which test can help determine the cause of Ms. T’s skin and soft tissue infection before the culture result is available?
2. What type of antibiotic is dicloxacillin and what is its mechanism of action?
3. Is dicloxacillin an appropriate drug for treatment of an MSSA skin infection?
4. What is the mechanism of action of vancomycin?
5. Why is vancomycin administered orally instead of intravenously to treat C. difficile infection?
BIOCHEMISTRY OF BACTERIAL CELL WALL SYNTHESIS
Cell Wall Structure and Function
Peptidoglycan, named for its peptide and sugar composition, is a three-dimensional meshwork of peptide-cross-linked sugar polymers that surrounds the bacterial cell just outside its cytoplasmic membrane (Fig. 35-1). Peptidoglycan is also known as murein, after the Latin murus (wall). Nearly all clinically important bacteria produce peptidoglycan. The major exceptions are Mycoplasma pneumoniae, which can cause atypical pneumonia, and the intracellular form (or “reticulate body”) of Chlamydia trachomatis, which can cause a sexually transmitted infection. Peptidoglycan is critically important for the survival of bacteria, which experience large fluctuations in osmotic pressure depending on their environment. The peptidoglycan meshwork wrapped around the cell provides the tensile strength required to withstand high turgor pressures that would otherwise cause the plasma membrane to rupture. Since peptidoglycan is essential for bacterial survival, its biosynthesis is a major target for antibiotics. The largest and most widely used class of bacterial cell wall synthesis inhibitors, the beta-lactam (β-lactam) antibiotics, inhibit the transpeptidase enzymes that mediate peptide cross-linking of the sugar polymers.
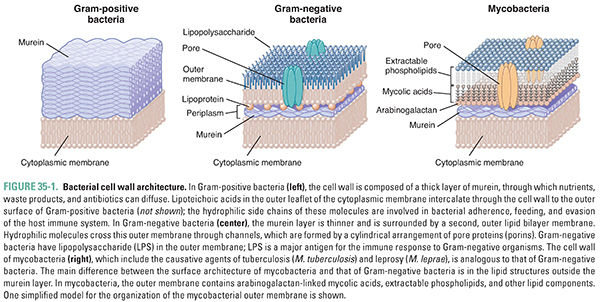
Bacteria are conventionally divided into two groups, Gram-positive and Gram-negative, based on the relative ability of the bacteria to retain the purple color of the gentian violet component of the Gram stain after being washed with an organic solvent such as acetone. Gram-positive bacteria retain the stain and appear purple, whereas Gram-negative bacteria lose the stain and appear pink from the subsequently applied safranin counterstain. Gram staining is frequently used to help identify the bacteria present in a specimen of body fluid such as urine, sputum, or pus. The Gram stain is one test that the urgent care clinician used to determine what type of organism was causing Ms. T’s skin abscess and cellulitis in the introductory case. The ability to retain Gram stain results from two distinguishing characteristics of cell wall architecture (Fig. 35-1). First, Gram-negative bacteria possess an outer membrane, an asymmetric bilayer in which the outer leaflet is composed of lipopolysaccharide. This structurally unusual membrane forms a permeability barrier that excludes a wide variety of molecules and limits the penetration of Gram stain into the periplasm, the space between the inner and outer membranes where the peptidoglycan layer is located. Second, Gram-positive bacteria have a very thick murein layer, whereas Gram-negative bacteria have only a thin layer. Since Gram stain binds to peptidoglycan, and the binding capacity and accessibility of the thick murein layer are much greater in Gram-positive than Gram-negative organisms, the Gram-positive bacteria stain purple.
The outer membrane of Gram-negative bacteria not only limits the penetration of Gram stain into the periplasm, but it also prevents the penetration of many other molecules, including antibiotics that target peptidoglycan synthesis—such as vancomycin and bacitracin. Hence, although Gram-negative organisms express the molecular targets for these antibiotics, they are not susceptible. To enable uptake of hydrophilic nutrients and excretion of hydrophilic waste products, Gram-negative bacteria have outer membrane porins—beta-barrel proteins that traverse the outer membrane and allow certain molecules to pass in and out (see Fig. 35-1). Porins are important pharmacologically because it is through these pores that most hydrophilic antibiotics with activity against Gram-negative organisms gain access to the murein layer and to the structures beneath this layer. Also important pharmacologically are the lipopolysaccharides (LPS) that compose the outer leaflet of the outer membrane of Gram-negative bacteria. Lipopolysaccharides are amphipathic molecules that protect bacteria from toxic hydrophilic host molecules such as bile salts. Lipopolysaccharides are also important for bacterial adherence to host cells and for evasion of the host immune response. Polymyxin is a topically used antibiotic that facilitates its own entry into the periplasm by binding to LPS and disrupting the integrity of the outer membrane. Once in the periplasm, polymyxin permeabilizes the inner membrane, discharging the membrane potential so that bacterial cells no longer generate the energy required for survival. Although polymyxin is too toxic for systemic use in people, its mechanism of action suggests that it may be possible to develop less toxic molecules that breach the outer membrane and allow the passage of antibiotics to their molecular targets in Gram-negative bacteria.
Gram-positive bacteria do not have an outer membrane; the extracellular enzymes involved in cell wall synthesis are therefore accessible to a wider range of antibiotics than those that can penetrate Gram-negative organisms. However, the cell wall of Gram-positive organisms is not composed simply of peptidoglycan; there is also a set of other cell wall polymers that play important roles in adherence to host tissue and other aspects of pathogenicity. These include lipoteichoic acids and wall teichoic acids, anionic polymers that are typically composed of acyclic sugar-phosphate repeats functionalized with D-alanine and cyclic sugars such as glucose. Lipoteichoic acids are anchored in the bacterial membrane and extend into the peptidoglycan layers. Wall teichoic acids are covalently attached to peptidoglycan and extend through and beyond its outermost layer. These polymers are important for bacterial infection of the host, and the pathways of teichoic acid biosynthesis are possible targets for antibiotics. In some Gram-positive organisms, including Staphylococcus aureus, the peptidoglycan layers are also functionalized with proteins that are required for pathogenesis. These proteins are covalently attached to uncross-linked peptides in peptidoglycan by enzymes called sortases. Sortases have also been suggested as possible targets for antibiotics.
These important structural differences between the cell envelopes of Gram-negative and Gram-positive bacteria lead to differential access of antibiotics to cellular targets and also present different opportunities for the development of new antibiotics. Despite this, peptidoglycan biosynthesis, which is conserved among Gram-negative and Gram-positive organisms, remains the most important antibacterial cell envelope target. In fact, the peptidoglycan biosynthetic pathway is one of a very small number of broad-spectrum antibacterial targets that exist in bacterial pathogens. The other broad-spectrum targets include DNA synthesis, RNA synthesis, and protein synthesis (see Chapter 34, Pharmacology of Bacterial Infections: DNA Replication, Transcription, and Translation). Of these processes, only peptidoglycan biosynthesis is unique to bacteria.
Peptidoglycan biosynthesis occurs in three major stages. The first stage is intracellular and involves the synthesis of murein monomers from amino-acid and sugar building blocks; the second and third stages involve the export of these murein monomers to the surface of the inner membrane, followed by their polymerization into linear peptidoglycan polymers and their cross-linking into two-dimensional lattices and three-dimensional mats (Fig. 35-2). Because the details of bacterial cell wall synthesis can be daunting, it is helpful to keep in mind the three major stages—monomer synthesis, glycan polymerization, and polymer cross-linking—in the discussion that follows. In principle, any of the biochemical steps in peptidoglycan biosynthesis could be a target for antibiotics; in practice, clinically used antibiotics target only a few of the steps in these stages. A vast number of secondary metabolites produced by soil and marine microorganisms also block peptidoglycan biosynthesis, providing a reservoir of structurally and functionally novel compounds for possible clinical development as our existing antibiotics fail due to the spread of resistance.
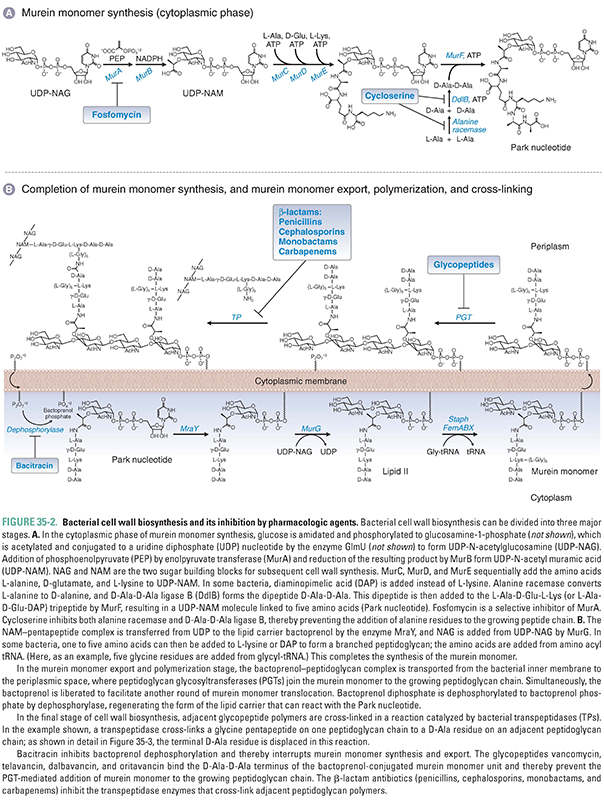
The murein monomer is a disaccharide comprising N-acetylglucosamine connected via a beta linkage to the C4 hydroxyl of N-acetyl muramic acid, which is functionalized on the C3 lactate moiety with a peptide (Fig. 35-2). The first phase of peptidoglycan synthesis takes place in the cytoplasm and involves the conversion of UDP-N-acetylglucosamine (UDP-NAG), a nucleotide-sugar used as a building block in many cell wall polymers, to UDP-N-acetyl muramic acid pentapeptide (UDP-NAM-peptide; also known as the Park nucleotide). The first two enzymes in this process, MurA and MurB, convert the C3 hydroxyl of NAG to lactate. MurA, also known as enolpyruvate transferase, transfers enolpyruvate from phosphoenolpyruvate (PEP) to UDP-NAG to form UDP-NAG pyruvate enol ether (Box 35-1). The flavoenzyme MurB (also known as UDP-NAG-enolpyruvate reductase) then reduces the double bond to produce UDP-NAM, which has a free carboxylate to serve as the handle for the peptide chain. UDP-NAM is a sugar unique to bacteria, and its biosynthesis thus provides opportunities for selective antibiotics. One clinically used antibiotic that blocks the biosynthesis of UDP-NAM is fosfomycin, a PEP analogue that inhibits MurA.
BOX 35-1 Enzymes of Cell Wall Biosynthesis | |
Like most enzymes, the enzymes of cell wall biosynthesis are known by multiple names. The Mur naming convention used here is the emerging standard, but the enzymes are also still known by the following descriptive names (among others): GlmU Diamine N-acetyltransferase MurA Enolpyruvate transferase MurB UDP–NAG-enolpyruvate reductase MurC UDP–NAM-L-Ala synthetase MurD UDP–NAM-L-Ala-D-Glu synthetase MurE UDP–NAM-L-Ala-D-Glu-2,6-diaminopimelate synthetase MurF UDP–NAM-tripeptide-D-Ala-D-Ala synthetase MraY UDP–NAM-pentapeptide:undecaprenol-phosphate transferase MurG Undecaprenoldiphospho-NAM-pentapeptide:NAG transferase Note: Undecaprenol is another name for bactoprenol. |
The peptide component of UDP-NAM-peptide is assembled on the C3 lactate from amino acids and dipeptides by a series of ATP-dependent ligases. MurC, MurD, and MurE sequentially add the amino acids L-alanine, D-glutamate, and a diamino acid—either L-lysine or diaminopimelic acid (DAP)—to UDP-NAM. DAP differs from lysine in having a carboxyl group as well as an amine on the side chain. Most Gram-positive bacteria use L-lysine, whereas a minority of Gram-positive and all known Gram-negative bacteria use DAP. This is noteworthy because m-DAP is not found in humans, and therefore, it offers a unique target for future drug development.
Peptide formation continues with the addition of a D-alanyl-D-alanine dipeptide (D-Ala-D-Ala) to the growing chain. The dipeptide is synthesized from two molecules of L-alanine in two reactions. Because amino acids are usually available in the environment in the L-conformation—which is the conformation found in most mammalian proteins—the first reaction requires the transformation of two molecules of L-alanine into D-alanine. This reaction is catalyzed by the enzyme alanine racemase. (Similarly, a glutamate racemase converts L-glutamate to D-glutamate to provide the building block for the second amino acid in the peptide chain.) In the second reaction, an ATP-dependent enzyme called D-Ala-D-Ala synthetase (or D-Ala-D-Ala ligase B [DdlB]) joins the two D-alanines together after first activating one as the AMP-ester. The resulting D-Ala-D-Ala dipeptide is added to the UDP-NAM-tripeptide by MurF to form UDP-NAM-L-Ala-D-Glu-L-Lys (or m-DAP-)-D-Ala-D-Ala, a molecule referred to as the Park nucleotide (Fig. 35-2A).
The second phase of peptidoglycan synthesis takes place on the inner surface of the cytoplasmic membrane and begins with the transfer of UDP-NAM-peptide to a phospholipid carrier embedded in the membrane (Fig. 35-2B). This carrier is called bactoprenol phosphate or, alternatively, undecaprenol phosphate in recognition of the fact that it is assembled from 11 five-carbon isoprene units. Bactoprenol phosphate (BP) is called a carrier because murein monomers as well as many other cell wall precursors are assembled on it, delivered by it to the surface of the plasma membrane, and then released in a process that regenerates the carrier for further cycles of reaction and precursor transport. The reaction by which UDP-NAM-peptide is anchored to the carrier lipid is mediated by an integral membrane protein called MraY. This enzyme catalyzes a diphosphate exchange reaction, so called because the uridine diphosphate linkage to the NAM-peptide is replaced with an undecaprenol diphosphate linkage, in a chemical exchange reaction illustrated in Figure 35-2B. This reaction is thermodynamically neutral since the products contain the same types of bonds as the starting materials, and indeed, MraY is a readily reversible enzyme. Once the NAM-peptide is anchored to the carrier lipid on the cytoplasmic surface of the membrane, a membrane-associated enzyme called MurG catalyzes the transfer of N-acetyl glucosamine to the C4 hydroxyl of the NAM sugar to produce a lipid-anchored NAM-NAG disaccharide commonly known as Lipid II. Finally, in some Gram-positive bacteria, including S. aureus, a linker peptide, typically composed of five glycine residues, is usually added to the lysine (or DAP) side chain amine. The additional amino acids in the branching peptide are not added in the same fashion as those in the main peptide chain. Rather than being activated as AMP-esters for attack by nucleophilic amines, these amino acids are activated by ester bonds to tRNA molecules.
In S. aureus, three different enzymes (FemA, FemB, and FemX) assemble the glycine pentapeptide branch from the appropriately charged tRNAs. FemX, which attaches the first glycine, is essential for survival. FemA and FemB (which add the next four glycines) are not essential for survival, but their deletion compromises the viability of the organism by affecting cross-linking and integrity of the cell wall. Thus, these enzymes are potential targets for antibiotic development. In Gram-negative bacteria, the murein monomers are usually cross-linked to one another directly, without the use of a branching peptide.
These steps complete the synthesis of a murein monomer. Before the final stages of cell wall synthesis can take place, the murine monomer must be transferred from the inner surface of the cytoplasmic membrane to the outer surface. How this is accomplished is an active area of investigation and may be a potential target for new antimicrobial agents.
Murein monomers on the external surface of the cytoplasmic membrane undergo polymerization to make long glycan chains through several rounds of glycosylation. Polymerization is catalyzed by enzymes called peptidoglycan glycosyltransferases (PGTs, or formerly, transglycosylases). These enzymes catalyze several rounds of elongation by addition of disaccharide subunits to the reducing end of the growing polymer without releasing it. With each glycosylation reaction, bactoprenol diphosphate is released and returns to the inner surface of the cytoplasmic membrane, where it loses a phosphate group to form bactoprenol phosphate; this step is catalyzed by a dephosphorylase. Bactoprenol phosphate is now ready to accept another Park nucleotide (Fig. 35-2B).
The PGTs are often found as N-terminal catalytic domains in bifunctional proteins that also have a C-terminal transpeptidation domain; however, they can also be found as monofunctional PGTs (known as MGTs). Most bacteria have a number of structurally related PGTs, some bifunctional and some monofunctional. Their enzymatic activities are similar in vitro, but they are presumed to play different roles in cells. For example, in rod-shaped organisms, some PGTs are dedicated to the synthesis of side wall peptidoglycan, whereas others are dedicated to the synthesis of septal peptidoglycan. Nevertheless, these enzymes can partially substitute for one another, complicating a detailed understanding of their specific roles. One possible way of understanding this biological complexity is that bacteria have evolved to have multiple overlapping systems to ensure survival should specific problems arise in an individual machine. This partial redundancy can be a disadvantage from the standpoint of antibiotic treatment.
In the final stage of cell wall synthesis, murein chains are cross-linked to one another by enzymes called transpeptidases (TPs). Because transpeptidases were first identified as the molecular targets of penicillin, they are also called penicillin-binding proteins (PBPs). The PGT domain couples murein monomers to produce glycan strands. These oligosaccharide chains must then be cross-linked through their stem peptides to produce the murein found in bacterial cell walls. The transpeptidation reaction takes place in two steps: activation and coupling. In the activation step, a serine hydroxyl in the active site of a TP enzyme attacks the D-Ala-D-Ala amide bond of one of the stem peptides on the glycan polymer, forming a covalent enzyme–peptidoglycan intermediate and releasing alanine. In the coupling step, a free amino group on the terminal amino acid of the interbridge peptide (glycine for many Gram-positive bacteria) or on DAP (Gram-negative bacteria) then attacks this intermediate, producing a new amide bond cross-link between the two stem peptides and regenerating the active enzyme (Figs. 35-2B and 35-3). Penicillin, a β-lactam, apparently mimics the terminal D-Ala-D-Ala substrate: it binds in the TP active site, where it then reacts with the serine nucleophile to form a covalent enzyme–penicillin complex (Fig. 35-3). This modification inactivates the enzyme, thereby resulting in lower degrees of cell wall cross-linking; in turn, this compromises the integrity of the cell wall and eventually causes cell lysis (see discussion below).
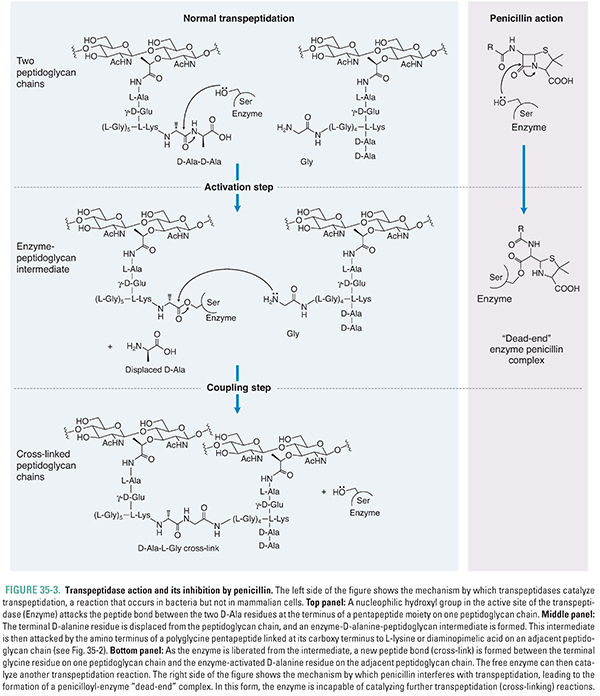
Bacteria typically contain several TPs with different but overlapping specificities. As described above for PGTs, these different enzyme isoforms are used to build different parts of the wall. Escherichia coli, for example, has six transpeptidases, some of which build the cylindrical middle of this rod-shaped bacteria, and others of which build its hemispherical ends. It is believed that differences in the number and type of cross-links and glycan chain length give each bacterial species its characteristic shape and size and the cell wall of each species its characteristic thickness. Consistent with this hypothesis, it has been found that the complement of transpeptidases differs from species to species and especially between rods such as E. coli and C. perfringens and spherical cocci such as streptococci and staphylococci.
It is thought that, in some cases, bacteria exploit the presence of multiple TPs to develop antibiotic resistance in the clinic. A major form of resistance develops in S. aureus when strains acquire a resistant TP that is capable of cross-linking peptidoglycan even when exposed to the β-lactam methicillin, which typically inactivates TPs in a manner similar to penicillin (see discussion below). The cell wall produced by methicillin-resistant S. aureus (MRSA) in the presence of drug has lower levels of cross-linking than in the absence of drug, which is presumed to be due to the inefficiency of the resistant TP. One possible strategy for overcoming MRSA is to further weaken the cross-linking ability of this resistant TP.
Mycobacterial Cell Wall Synthesis
The cell wall structures described above are found in the vast majority of clinically relevant bacteria, including Gram-positive cocci such as streptococci and staphylococci, Gram-negative rods such as E. coli and Pseudomonas aeruginosa, and Gram-positive rods such as C. perfringens. However, a discussion of cell wall structure would not be complete without mentioning the unusual cell envelopes of the Corynebacteriae, a group of bacteria that includes the important pathogens Mycobacterium tuberculosis and Mycobacterium leprae. These bacteria are classified as high G+C (i.e., a high percentage of guanine and cytosine in their DNA) Gram-positives, but their cell envelopes have characteristics of both Gram-positive and Gram-negative bacteria.
Unlike other Gram-positives, the Corynebacteriae have an outer membrane. The NAM sugars in the peptidoglycan layer that surrounds the cytoplasmic (inner) membrane have covalently attached NAG-arabinogalactan polymers to which are attached mycolic acids. The mycolic acids have long alkyl chains containing as many as 90 carbons, and these alkyl chains form a waxy layer that make the bacteria resistant to acid decolorization (acid-fast). The mycolic acids are essential for the assembly of the outer membrane, but the organizational details are unclear. In addition to mycolic acids, the outer membrane of mycobacteria contains secreted phospholipids called extractable lipids (see Fig. 35-1). Mycobacteria have outer membrane porins, but their structures are different from the structures of the porins found in Gram-negative bacteria.
The synthesis of NAG-arabinogalactan begins with the transfer of a molecule of NAG phosphate from UDP-NAG to mycobacterial bactoprenol phosphate. Next, a molecule of the sugar rhamnose is added, followed by the addition of the several galactose and arabinose units that make up arabinogalactan. Arabinosyl transferase catalyzes the addition of the arabinose units. Mycolic acid is a long, complex, branched fatty acid. The starting materials for its synthesis include long, saturated hydrocarbon chains that are synthesized from two-carbon units carried by acetyl CoA. The enzyme fatty acid synthetase 1 (FAS1) catalyzes the formation of these saturated hydrocarbon chains, and the enzyme fatty acid synthetase 2 (FAS2) catalyzes the linkage of these chains. The linked product then undergoes several enzymatic transformations to become mycolic acid. Mycolic acid is eventually added to NAG-arabinogalactan, which, in turn, is attached to NAM to organize and form a major component of the mycobacterial outer membrane (Figs. 35-1 and 35-4). In principle, any step in this process is susceptible to pharmacologic intervention. As discussed below, standard antimycobacterial treatment regimens include antibiotics that target both the synthesis of NAG-arabinogalactan and the early reactions of mycolic acid synthesis.
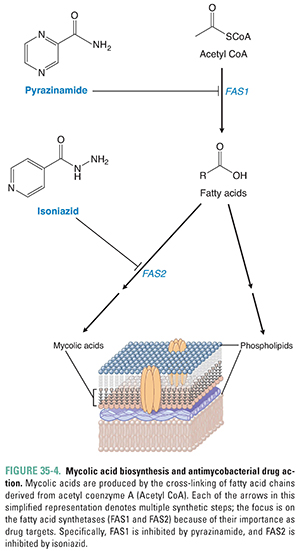
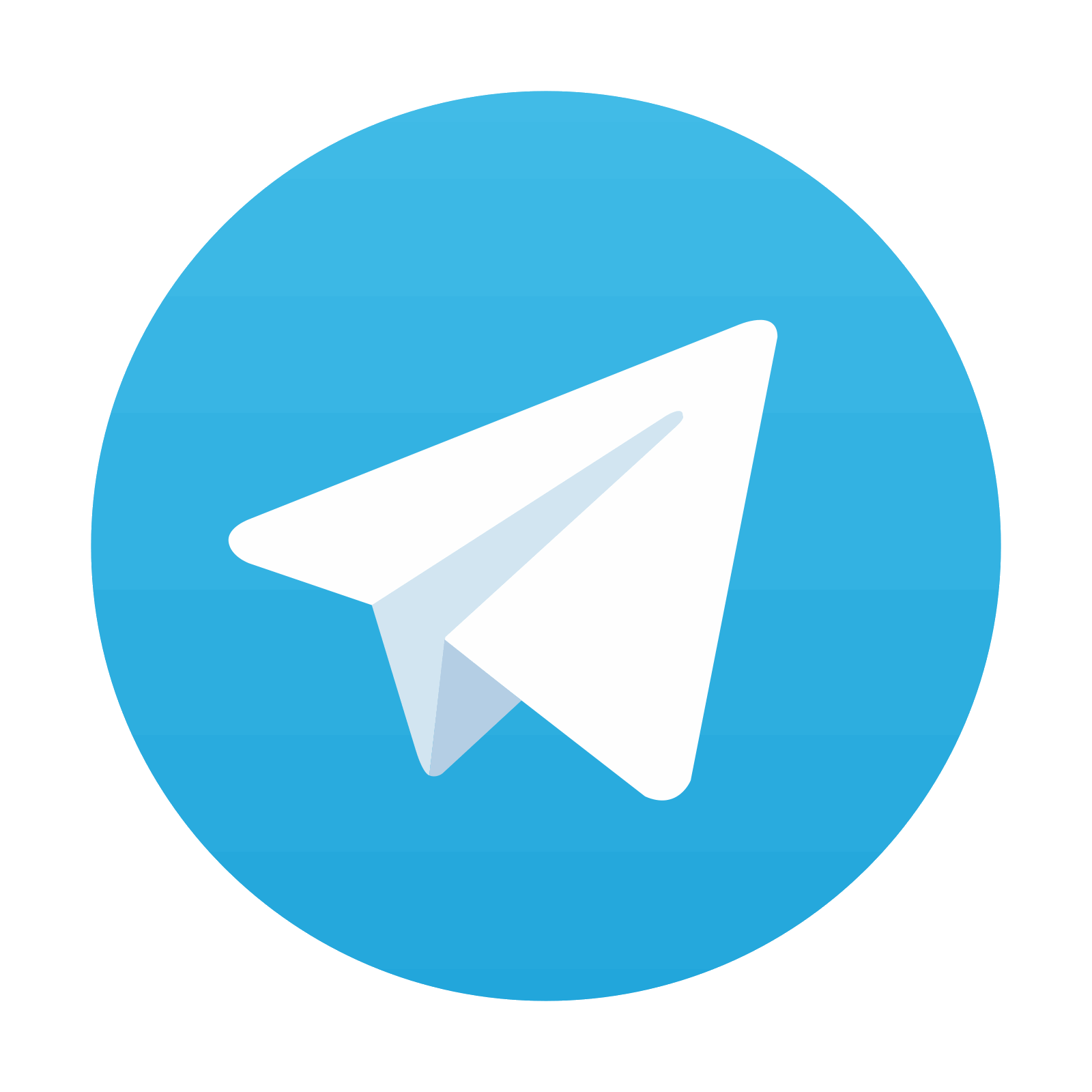
Stay updated, free articles. Join our Telegram channel
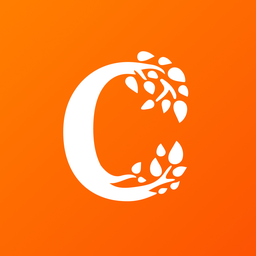
Full access? Get Clinical Tree
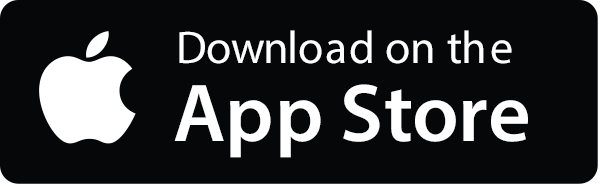
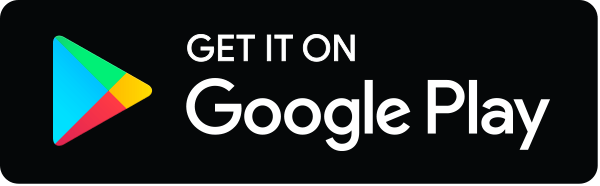