Gene symbol
Gene product
Chromosomal location
Key information
Definitive disease-causing genes (causative mutations of early onset Alzheimer disease )
APP (AD1)
Amyloid precursor protein
21q21.3
Autosomal dominant, 25 pathogenic mutations, 16 % of early onset AD
PSEN1 (AD3)
Presenilin-1
14q24
Autosomal dominant, 185 pathogenic mutations, 66 % of early onset AD
PSEN2 (AD4)
Presenilin-2
1q31
Autosomal dominant, 12 pathogenic mutations
Genes with increased susceptibility (risk variants for late-onset Alzheimer disease )
APOE (AD2)
Apolipoprotein E
19q13.32
SORL1
Sortilin-related receptor
11q24.1
ABCA7
ATP-binding cassette, subfamily A, member 7
19p13.3
BIN1
Bridging integrator 1
2q14.3
CD33 (SIGLEC6)
CD33 antigen/Sialic-acid binding immunoglobulin-like lectin 6
19q13.41
CD2AP
CD2-associated protein
6p12.3
CLU
Clusterin
8p21.1
CR1
Complement component receptor 1
1q32.2
EPHA1
Ephrin receptor EphA1
7q34-q35
MS4A4E/MS4A6A
Membrane-spanning 4-domains, ubfamily A, members 6E, 4A
11q12.2
PICALM
Phosphatidylinositol-binding clathrin assembly protein
11q14.2
Mutations in presenilin-1 (PSEN1), located at chromosomal position 14q24.3, are responsible for the highest percentage of autosomal dominant EOFAD. Approximately 185 pathogenic mutations have been identified to date [3], all with complete penetrance by age 60–65 years. Presenilin-1 is the catalytic component of γ-secretase, a protein complex responsible for the cleavage of membrane proteins, including APP. Normal γ-secretase activity yields mainly Aβ1–40 with smaller amounts of Aβ1–42. PSEN1 mutations alter the secretase activity [4], which likely increases the ratio of Aβ1–42 to Aβ1–40, facilitating the deposition of amyloidogenic species. Presenilin-2 (PSEN2), located at chromosomal position 1q31-q42, is highly homologous to PSEN1 and participates in the γ-secretase complex as the catalytic domain in the absence of Presenilin-1. PSEN2 mutations are less common than PSEN1 variants, with only 13 pathogenic mutations identified to date [3]. Compared to PSEN1, patients with PSEN2 mutations tend to have an older age of onset (accounting for the very small number of LOAD caused by an inherited mutation), longer course of disease, and more variable penetrance.
The association of the apolipoprotein E (APOE) ε4 allele with LOAD in non-Hispanic whites of European ancestry has been well known for more than a decade. ApoE is a plasma protein involved in the transport of cholesterol that exists as three isoforms determined by three alleles (ε2, ε3, and ε4). The ApoE ε2 and ε3 alleles are the most common in the general population. A single ApoE ε4 allele conveys a two-fold to three-fold increased risk of developing AD, whereas having two copies is associated with a five-fold increased risk, demonstrating an additive risk association. The ε2 allele is considered protective, also in an additive manner, so that a homozygous ε2/ε2 genotype confers a lower risk than just one ε2 allele. The ε4 allele s associated with not only a higher risk of developing the disease, but also an earlier age of onset; however, the presence of ε4 is neither sufficient nor necessary to develop AD, since approximately 45 % of AD patients do not have an ε4 allele [1]. Other susceptibility genes associated with increased risk for AD are shown in Table 18.1.
Clinical Utility of Testing
The use of APOE genotyping in the clinical setting remains controversial. As a diagnostic adjunct in the clinical evaluation of a patient with late-onset dementia, the test may have some clinical utility; however, there is general agreement that this test should not be used for predictive purposes and should never be considered for prenatal testing. In contrast, within the context of a high degree of clinical suspicion and the documentation of a family history of AD, clinical molecular testing for APP, PSEN1, and PSEN2 has demonstrable utility. Confirmation of a pathologic mutation in a proband will establish a diagnosis and clarify the EOFAD subtype such that both presymptomatic testing for appropriate family members and prenatal testing for at-risk pregnancies could be offered to family members.
Available Assays
APOE genotyping can be performed by traditional polymerase chain reaction-restriction fragment length polymorphism (PCR-RFLP) analysis, Sanger sequencing, or TaqMan® SNP assay (Life Technologies, Grand Island, NY). Detection of pathologic mutations in APP, PSEN1, and PSEN2 requires DNA sequencing, either Sanger sequencing or next-generation sequencing.
Interpretation of Test Results
Interpretation of an APOE genotype requires the concurrent evaluation of clinical information available at the time of testing and should never be interpreted in the absence of this information. As such, testing is limited to situations where the index of clinical suspicion for AD is quite high. In contrast, the identification of a pathologic PSEN1 or PSEN2 mutation in a symptomatic individual is diagnostic. While both presymptomatic as well as prenatal testing are theoretically possible in mutation-positive kindred, the possible existence of incomplete or reduced penetrance must be discussed during genetic counseling.
Laboratory Issues
APOE genotyping is commercially available in several reference laboratories. The College of American Pathologists (CAP) offers APOE genotyping proficiency testing challenges twice a year as part of the CAP/ACMG Biochemical and Molecular Genetics Survey. Methods-based sequencing challenges (Sanger or next-generation sequencing) are also available through CAP.
Parkinson Disease
Molecular Basis of Disease
Parkinson disease (PD) is a chronic, progressive, idiopathic neurodegenerative disorder of late onset, clinically characterized by the development of parkinsonism, which consists of rigidity, bradykinesia, resting tremor, and postural instability. Additional frequent non-motor symptoms include insomnia, depression, anxiety, rapid eye movement, behavior disorder, fatigue, constipation, dysautonomia, and anosmia. With progression of the disease, 15 % of affected individuals develop psychosis (visual hallucinations and delusions) and dementia. PD is the second most common neurodegenerative disorder, after AD [5]. The neuropathologic hallmark is the presence of α-synuclein positive intracytoplasmic inclusions called Lewy bodies, with selective degeneration of dopaminergic neurons in the pars compacta of the substantia nigra in the midbrain. Although most patients present with a late-onset sporadic form of the disease, the identification of familial forms of PD has clearly established the role of genetic factors in disease etiology and pathogenesis. Of note, the neuropathology of some forms of genetically based parkinsonism may differ significantly from the classic findings documented in classical PD, including nigral pathology in the absence of Lewy bodies [6].
Genes for ten familial forms of PD (PARK1, PARK2, PARK4, PARK6, PARK7, PARK8, PARK9, PARK12, PARK15, and PARK17) have been identified (Table 18.2). The inheritance patterns for PD include autosomal dominant (PARK1, PARK4, PARK8, and PARK17), autosomal recessive (PARK2, PARK6, PARK7, PARK9, and PARK15), and X-linked (PARK12). Three different but interconnected cellular processes appear to be involved in these forms: synaptic transmission, mitochondrial quality control, and lysosome-mediated autophagy [7].
Table 18.2
Molecular genetic classification of Parkinson disease (PD)
Locus name | Gene | Gene product | Chromosomal location | Inheritance pattern |
---|---|---|---|---|
PARK1, PARK4 | SNCA | Alpha-synuclein | 4q21 | Autosomal dominant |
PARK2 | PARK2 | Parkin | 6q25 | Autosomal recessive |
PARK6 | PINK1 | Serine/threonine protein kinase PINK1 | 1p35 | Autosomal recessive |
PARK7 | PARK7 | Protein DJ-1 | 1p36 | Autosomal recessive |
PARK8 | LRRK2 | Leucine-rich repeat serine/threonine protein kinase 2 | 12p11 | Autosomal dominant |
PARK9 | ATP13A2 | Probable cation-transporting ATPase 13A2 | 1p36 | Autosomal recessive |
PARK12 | TAF1 | Transcription initiation factor TFIID subunit 1 | Xq21-q25 | X-linked |
PARK15 | FBX07 | F-box only protein 7 | 22q12.3 | Autosomal recessive |
PARK17 | VPS35 | Vacuolar protein sorting-associated protein 35 | 16q11.2 | Autosomal dominant |
PARK1 is associated with early-onset autosomal dominant PD. Despite an earlier age of onset, PARK1 patients have typical dopa-responsive parkinsonism symptoms with classic neuropathologic findings at autopsy. Three pathogenic missense mutations in the α-synuclein (SNCA) gene at 4q21 have been identified. The first, A53T, was found in 13 families of Italian–Greek descent [8] and the second, A30P, identified in a single German kindred [9]. A third point mutation, E46K, was described in a Spanish kindred with clinical manifestations of both parkinsonism and dementia, clinically similar to Lewy body dementia [10]. All three point mutations appear to be highly penetrant. Other pathogenic variants include gene duplications and triplications [3], some of which are responsible for the PARK4 phenotype with late-onset parkinsonism and extensive cortical Lewy bodies. α-Synuclein is a 140 amino acid protein predominantly expressed in neuronal tissues. Although the function of α-synuclein is not well characterized, this protein is one of the major protein constituents of the Lewy body. One attractive hypothesis proposes that the mutant forms of the protein have a propensity to oligomerize and form toxic neuronal aggregates that contribute to the formation of insoluble fibrils through the disruption of the cellular ubiquitin–proteasome pathway and ultimately neuronal cell death via an apoptotic mechanism.
PARK2, or juvenile-onset autosomal recessive PD, is associated with mutations in the PARK2 gene that maps to chromosome 6q25. The gene consists of 12 exons and encodes a 465 amino acid protein, parkin. Functionally, parkin contains an ubiquitin-like domain in the N-terminus and two RING finger domains in the C-terminus. Similar to other proteins containing RING finger domains, parkin has been shown to have an E3 ubiquitin ligase activity. The association of parkin mutations with an autosomal recessive form of PD suggests that it is the loss of E3 ubiquitin ligase activity that directly contributes to the pattern of neurodegeneration seen in PD. Like PARK1, this mechanism is thought to involve disruption of the ubiquitin–proteasome system, resulting in the abnormal accumulation of substrate proteins. To date, more than 127 pathologic loss-of-function PARK2 mutations have been identified [7]. Mutations are generally exon rearrangements leading to deletions, duplications, triplications, or point mutations.
PARK6 is associated with mutations in the serine/threonine protein kinase gene (PINK1), with an autosomal recessive pattern of inheritance [5]. PARK7, another autosomal recessive form of PD, is caused by mutations (deletion and point mutations) in the PARK7 gene. PARK9 defines a particular autosomal recessive phenotype with parkinsonism and iron accumulation in basal ganglia and is due to mutations in the cation-transporting ATPase 13A2 protein encoded by ATP13A2. PARK15, also known as pallido-pyramidal syndrome, is characterized by the presence of pyramidal tract signs in addition to parkinsonism, and is associated with mutations in FBX07 [11].
PARK8 is a dominantly inherited late-onset form of PD associated with mutations in the leucine rich repeat kinase 2 gene (LRRK2). Nearly a dozen different pathogenic variants have been reported in LRRK2; the most common, p.Gly2019Ser, has been found in approximately 5–7 % of autosomal dominant PD. Of note, the frequency of this variant is substantially higher among individuals of Ashkenazi Jewish ancestry or Northern African ancestry [5].
Mutations of TAF1 are the only known cause of PD with X-linked inheritance (PARK12), a rare event only found among individuals of Panayan (Philippines) origin [5].
Clinical Utility of Testing
Testing for SNCA, PARK2, PINK1, PARK7, and LRRK2 mutations is available clinically. With a high degree of clinical suspicion and the documentation of a family history of PD, molecular testing has demonstrable utility. Confirmation of a pathologic mutation in a proband will establish a diagnosis and clarify the mode of inheritance such that both presymptomatic testing for appropriate family members and prenatal testing for at-risk pregnancies could be offered.
Available Assays
Testing for most of the PD genes can be performed by conventional Sanger sequencing of the mutation-containing exons. Testing for PARK2 mutations generally necessitates the use of a semiquantitative PCR methodology to detect heterozygous exonic rearrangements (deletions, duplications, and triplications), which would not be detected by conventional non-quantitative PCR or Sanger sequencing [12]. Next-generation sequencing panels of multiple Parkinson-associated genes are available in international laboratories.
Interpretation of Results
The identification of a pathologic mutation in a symptomatic proband is diagnostic.
Laboratory Issues
No commercial in vitro diagnostic test kits or formal proficiency testing are currently available.
Amyotrophic Lateral Sclerosis and Frontotemporal Degeneration
Molecular Basis of Disease
Amyotrophic lateral sclerosis (ALS) is a progressive neurodegenerative condition affecting upper and lower motor neurons characterized by rapidly progressive weakness, hyperreflexia, spasticity, muscle wasting, and fasciculations, usually leading to death in a few years. The peak incidence of ALS is in the sixth decade for sporadic cases (SALS, 90 %), and 10 years earlier for the 5–10 % of familial cases (FALS) [13].
Frontotemporal degeneration (FTD) is a neurodegenerative disorder characterized clinically by progressive changes in social, behavioral, and/or language function and pathologically by degeneration of the frontal and/or anterior temporal lobes. Clinical and pathologic phenotypes of FTD are heterogeneous, with several subtypes recognized depending on the most prominent clinical manifestation. In contrast to AD, memory is relatively preserved in FTD until late stages of disease. FTD accounts for 5–15 % of all dementia and is the second most common cause in the presenile age group. A subset of patients with FTD have associated motor neuron disease or parkinsonism. Onset is usually between 35–75 years of age and a family history of a similar disease is present in approximately 40 % of patients.
About 5 % of patients with ALS also have clinically documented FTD, and up to 30–50 % have milder evidence of executive dysfunction, supporting the hypothesis that at least in a majority of cases, FTD and ALS are part of a disease continuum. The presence of pathological deposits of TDP-43 in most cases of ALS, in most cases with overlap between FTD and ALS (FTD-ALS), and in most cases of FTD provides a potential unifying mechanistic link [14]. Mutations in the gene coding for TDP-43 (TARDBP) cause FALS in a 1–4 % of families.
The first gene to be identified in ALS is associated with the ALS1 locus. The SOD1 gene encodes copper-zinc superoxide dismutase, a metalloenzyme consisting of 153 amino acids with both copper and zinc binding sites. SOD1 catalyzes the conversion of superoxide anions to hydrogen peroxide and molecular oxygen and, as such, is thought to have a protective function in the cell by preventing oxidative damage caused by the accumulation of free radicals. More than 100 mutations have been reported in the SOD1 gene, with one, A5V (previously known as A4V), accounting for approximately 50 % of all mutations found in North American families. Approximately 20 % of patients with FALS and 3 % of patients with SALS have SOD1 mutations [13]. Incomplete penetrance has been documented in some families. Table 18.3 includes the gene products, chromosomal location, and clinical features of other ALS-related genes.
Table 18.3
Molecular genetic classification of amyotrophic lateral sclerosis (ALS) and frontotemporal lobar degeneration (FTD)
Locus name | Gene | Gene product | Chromosomal location | Inheritance pattern | Clinical presentation |
---|---|---|---|---|---|
ALS1 | SOD1 | Superoxide dismutase (Cu-Zn) | 21q22.1 | Autosomal dominant | 20 % of FALS |
ALS2 | ALS2 | Alsin | 2q33 | Autosomal recessive | |
ALS3 | ALS3 | Unknown | 18q21 | Autosomal dominant | FALS |
ALS4 | SETX | Senataxin | 9q34 | Autosomal dominant | Juvenile motor neuropathy with pyramidal features |
ALS5 | ALS5 | Unknown | 15q15 | Autosomal recessive | Juvenile ALS |
ALS6 | FUS/TLS | RNA-binding protein FUS | 16p11.2 | Autosomal recessive | ALS with or without FTD |
ALS7 | ALS7 | Unknown | 20p13 | Autosomal dominant | Incomplete penetrance |
ALS8 | VAPB | Vesicle-associated membrane protein-associated protein B/C | 20q13.32 | Autosomal dominant | Finkel type SMA |
ALS9 | ANG | Angiogenin | 14q11.2 | Autosomal dominant | FALS |
ALS10 | TARDBP | TDP-43 | 1p36.22 | Autosomal dominant | 1–4 % of FALS; ALS with or without FTD, FTD |
ALS 11 | FIG4 | Sac domain containing inositol phosphatase 3 | 6q21 | Autosomal dominant | ALS, Yunis–Varon syndrome, CMT 4J |
ALS12 | OPTN | Optineurin | 10p13 | Autosomal recessive | FALS |
ALS13 | ATXN2 | Ataxin-2 | 12q24.12 | Autosomal dominant | SCA2 |
ALS14 | VCP | Valosin-containing protein | 9p13.3 | Autosomal dominant | ALS with or without FTD, IBMPFD |
ALS15 | UBQLN2 | Ubiquilin-2 | Xp11.21 | X-linked | ALS with or without FTD |
ALS17 | CHMP2B | Chromatin-modifying protein 2B | 3p11.2 | Autosomal dominant | FALS/familial FTD |
ALS18 | PFN1 | Profilin-1 | 17p13.2 | Autosomal dominant | FALS |
C9FTD/ALS | C9orf72 | Uncharacterized protein C9orf72 | 9p21.2 | Autosomal dominant | Noncoding GGGGCC hexanucleotide repeat, 23–30 % of FTD/ALS |
CHCHD10- ALS/FTD | CHCHD10 | Coiled-coil-helix-coiled-coil-helix domain-containing protein 10, mitochondrial | 22q11.23 | Autosomal dominant | ALS, mitochondrial myopathy, SMA Jokela type |
FTD-MAPT | MAPT | Tau protein | 17q21 | Autosomal dominant | FTD |
FTD-GRN | GRN | Progranulin | 17q21 | Autosomal dominant | FTD |
Several different genes and chromosomal loci have been associated with FTD, primarily with an autosomal dominant inheritance. Mutations in the microtubule (MT)-associated protein tau (MAPT) are responsible for 10–20 % of familial cases, and all these have tau-based neuropathology. The main function of these proteins is to stabilize the MTs and to promote MT assembly by binding to tubulin. More than 40 mutations in MAPT have been associated with FTD [3]. These cause disease by either altering the MT-binding properties of tau or by altering the splicing of exon 10 of MAPT, which alters the ratio of isoforms with 3 or 4 microtubule binding repeats.
A number of other FTD families with linkage to the same region on chromosome 17 as MAPT (17q21) but with no identifiable MAPT mutation and no significant tau pathology demonstrate mutations in the progranulin (GRN) gene, including frameshift, nonsense, and splice-site mutations. Progranulin (PGRN) is a 593 amino acid-secreted growth factor known to play a role in central nervous system development. The underlying disease mechanism is likely due to loss-of-function leading to protein haploinsufficiency, and the end point is TDP-43 neuropathology. GRN mutations account for approximately 20 % of familial FTD.
In 2011, GGGGCC hexanucleotide repeat expansions in the first intron of the C9orf72 gene were identified as the most common genetic cause of both FTD and ALS [15, 16]. Normal individuals have between 2 and 32 GGGGCC repeats, whereas affected individuals with expansions usually have more than several hundred repeats [17]. The average mutation frequencies in North Americans and Europeans are 37 % for FALS, 6–7 % for SALS, 21–25 % for familial FTD, and 6 % for sporadic FTD (http://www.omim.org/entry/614260). Penetrance of the mutation is high, with 90 % of people with an expansion having onset of symptoms by age 70 years. No clear relationship between phenotype and expansion size has been determined. ALS patients with a C9orf72 expansion have earlier age of onset and reduced survival compared to ALS patients without an expansion.
Clinical Utility of Testing
Within the context of a high degree of clinical suspicion and the documentation of a positive family history, clinical molecular testing for most of the above ALS and FTD related genes has demonstrable utility. Confirmation of a pathologic mutation in a proband will establish a diagnosis and clarify the mode of inheritance such that both presymptomatic testing for appropriate family members and prenatal testing for at-risk pregnancies could be offered. Clinical testing for a C9orf72 hexanucleotide repeat expansion can help to confirm the diagnosis of ALS or FTD and be used for screening and genetic counseling of patients and family members after an affected family member has been found to carry an expansion.
Available Assays
Direct DNA sequencing analysis is most commonly used for ALS related genes (SOD1, ALS2, etc.), as well as for GRN and MAPT. When the mutation is known in the proband, directed sequencing is preferred in the screening of family members. Next-generation sequencing as well as Sanger sequencing panels that include many of these genes are currently available. The hexanucleotide expansion in C9orf72 uses a combination of PCR-based amplification for normal alleles with expansion analysis, usually by Southern blot analysis, for homozygous PCR results, and is available at most reference laboratories.
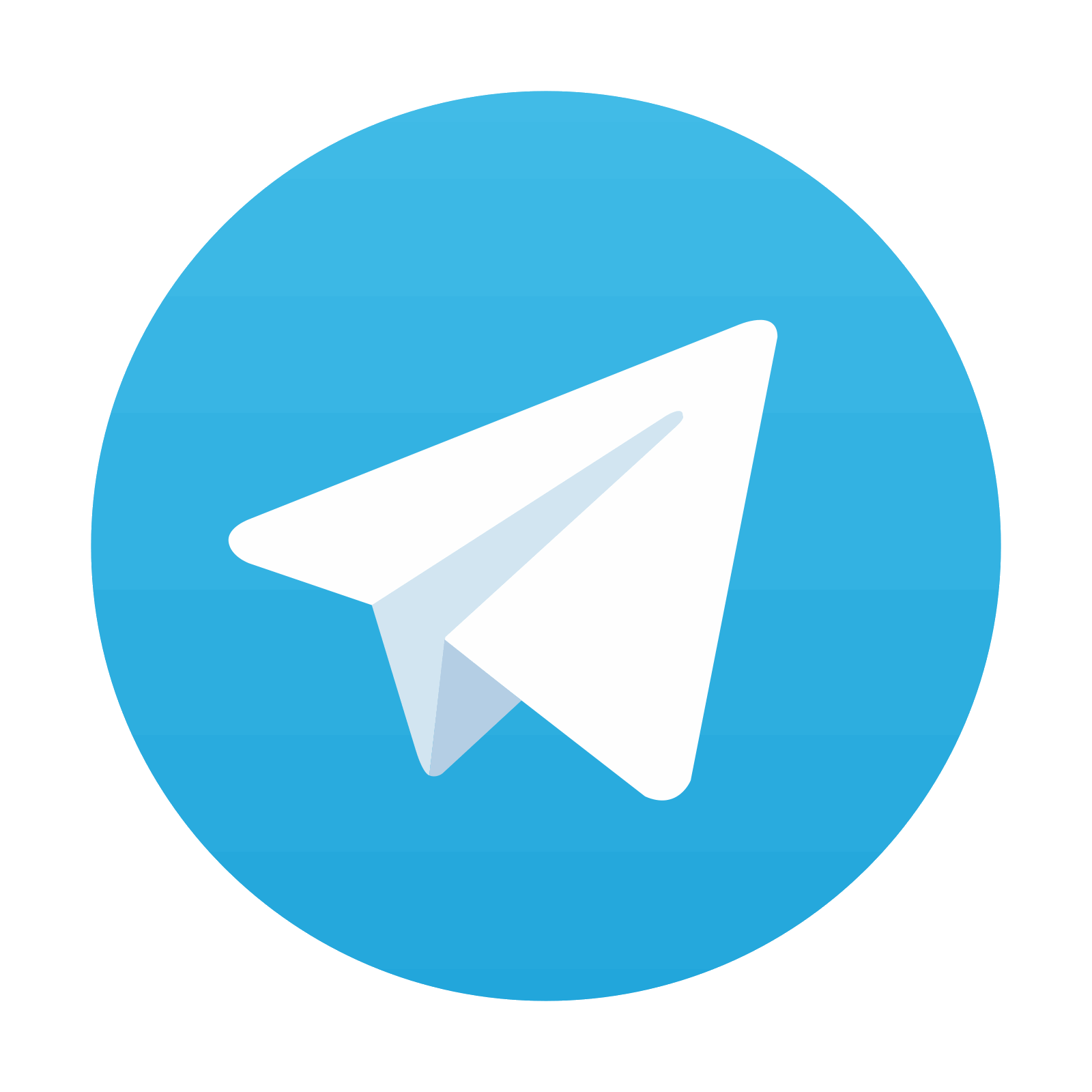
Stay updated, free articles. Join our Telegram channel
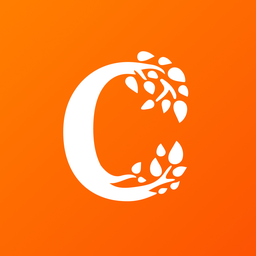
Full access? Get Clinical Tree
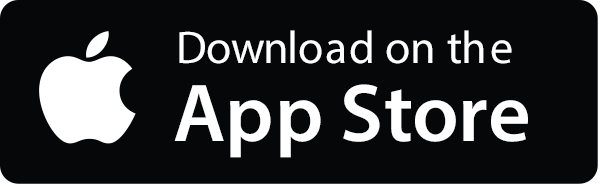
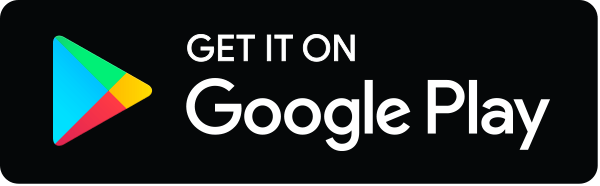