Chapter 21
Mechanisms of Hormonal Regulation
Valentina L. Brashers, Robert E. Jones and Sue E. Huether
The endocrine system is composed of various glands located throughout the body (Figure 21-1). These glands are capable of synthesizing and releasing special chemical messengers called hormones. The endocrine system has five general functions:
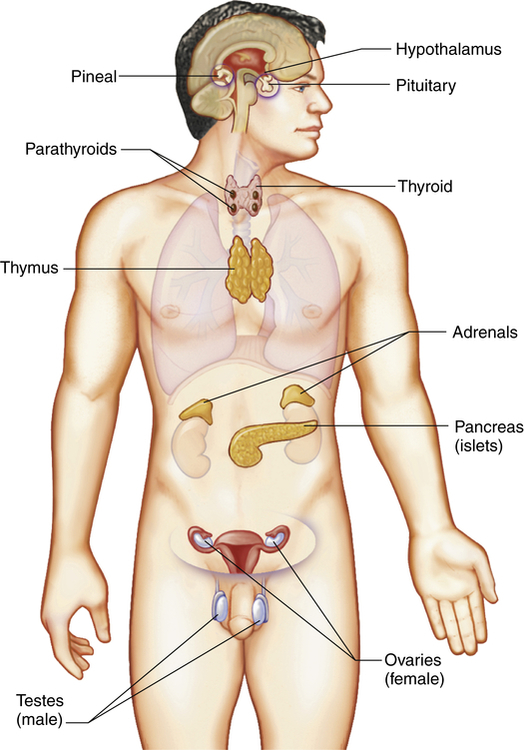
1. Differentiation of the reproductive and central nervous systems in the developing fetus
2. Stimulation of sequential growth and development during childhood and adolescence
3. Coordination of the male and female reproductive systems, which makes sexual reproduction possible
4. Maintenance of an optimal internal environment throughout the life span
5. Initiation of corrective and adaptive responses when emergency demands occur
Mechanisms of Hormonal Regulation
1. Hormones have specific rates and rhythms of secretion. Three basic secretion patterns are: (1) circadian or diurnal patterns, (2) pulsatile and cyclic patterns, and (3) patterns that depend on levels of circulating substrates (e.g., calcium, sodium, potassium, or the hormones themselves).
2. Hormones operate within feedback systems, either positive or negative, to maintain an optimal internal environment.
3. Hormones affect only cells with appropriate receptors and then act on those cells to initiate specific cell functions or activities.
4. Steroid hormones are either excreted directly by the kidneys or metabolized (conjugated) by the liver, which inactivates them and renders the hormone more water soluble for renal excretion. Peptide hormones are catabolized by circulating enzymes and eliminated in the feces or urine.
Hormones may be classified according to their structure, gland of origin, effects, or chemical composition. (Table 21-1 categorizes hormones based on structure.) The secretion and mechanisms of action of hormones represent an extremely complex system of integrated responses. Although much has been learned about these complex systems, many of the specific mechanisms of action are not yet understood. The endocrine and nervous systems work together to regulate responses to the internal and external environments.
TABLE 21-1
STRUCTURAL CATEGORIES OF HORMONES
STRUCTURAL CATEGORY | EXAMPLES |
Water Soluble | |
Peptides | Growth hormone |
Insulin | |
Leptin | |
Parathyroid hormone | |
Prolactin | |
Glycoproteins | Follicle-stimulating hormone |
Luteinizing hormone | |
Thyroid-stimulating hormone | |
Polypeptides | Adrenocorticotropic hormone |
Antidiuretic hormone | |
Calcitonin | |
Endorphins | |
Glucagon | |
Hypothalamic hormones | |
Lipotropins | |
Melanocyte-stimulating hormone | |
Oxytocin | |
Somatostatin | |
Thymosin | |
Thyrotropin-releasing hormone | |
Amines | Epinephrine |
Norepinephrine | |
Lipid Soluble | |
Thyroxine (an amine but lipid soluble) | Thyroxine (both thyroxine [T4] and triiodothyronine [T3]) |
Steroids (cholesterol is a precursor for all steroids) | Estrogens Glucocorticoids (cortisol) |
Mineralocorticoids (aldosterone) | |
Progestins (progesterone) | |
Testosterone | |
Derivatives of arachidonic acid (autocrine or paracrine action) | Leukotrienes Prostacyclins Prostaglandins Thromboxanes |
Regulation of Hormone Release
Feedback systems provide precise monitoring and control of the cellular environment. The most common feedback system, negative feedback, occurs because the changing chemical, neural, or endocrine response to a stimulus negates the initiating change that triggered the release of the hormone. An example of hormone negative feedback is shown in Figure 21-2, A. Thyroid-stimulating hormone (TSH) secretion from the anterior pituitary is stimulated by thyrotropin-releasing hormone (TRH) from the hypothalamus. Secretion of TSH stimulates the synthesis and secretion of thyroid hormones. Increasing levels of T4 (thyroxine) and T3 (triiodothyronine) then generate negative feedback on the pituitary and hypothalamus to inhibit TRH and TSH synthesis.
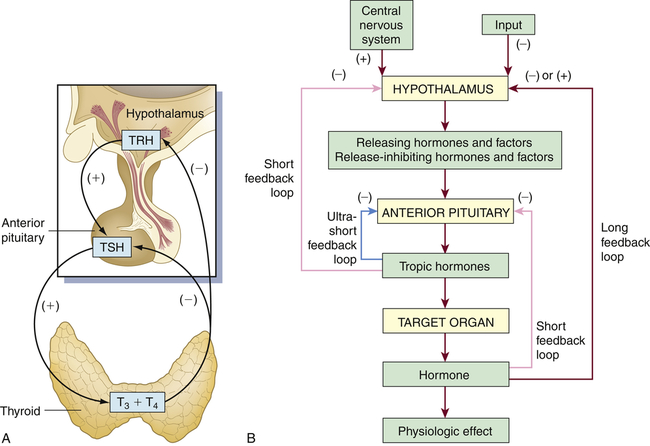
A, Endocrine feedback loops involving the hypothalamus-pituitary gland and end organs; in this example, the feedback loops for the thyroid gland (endocrine regulation). B, General model for control and negative feedback to hypothalamic-pituitary target organ systems. Negative-feedback regulation is possible at three levels: target organ (ultrashort feedback), anterior pituitary (short feedback), and hypothalamus (long feedback). TRH, Thyrotropin-releasing hormone; TSH, thyroid-stimulating hormone; T3, triiodothyronine; T4, tetraiodothyronine (thyroxine).
Negative-feedback systems are important in maintaining hormone concentrations within physiologic ranges. The lack of negative-feedback inhibition on hormonal release often results in pathologic conditions. As discussed in Chapter 22, various hormonal imbalances and related conditions are caused by excessive hormone production, which is the result of failure to “turn off” the system. These negative-feedback regulatory systems are diagrammed in Figure 21-2, B.
Hormone Transport
Once hormones are released into the circulatory system, they are distributed throughout the body. Peptide or protein hormones (pituitary, hypothalamic, and parathyroid hormones; and insulin) are water soluble and circulate in free (unbound) forms. Water-soluble hormones generally have a short half-life because they are catabolized by circulating enzymes. For example, insulin has a half-life of 3 to 5 minutes and is catabolized by insulinases. Lipid-soluble hormones, such as cortisol and adrenal androgens, are transported bound to a carrier or transport protein (Table 21-2) and can remain in the blood for hours to days. Only free hormones (those not bound to the carrier protein) can signal a target cell. Because an equilibrium exists between the concentrations of free hormones and hormones bound to plasma proteins, a significant change in the concentration of binding proteins can affect the concentration of free hormones in the plasma (see Table 21-2). (Mechanisms of hormone binding are discussed in Chapter 1.)
TABLE 21-2
BINDING PROTEINS, THEIR HORMONES, AND VARIABLES THAT AFFECT THEIR CIRCULATING LEVELS
BINDING PROTEIN | HORMONE | FACTORS THAT INCREASE BINDING PROTEIN LEVELS | FACTORS THAT DECREASE BINDING PROTEIN LEVELS |
Corticosteroid-binding globulin | Cortisol | Estrogen | Liver disease |
Progesterone | |||
Sex hormone–binding globulin | Dihydrotestosterone | — | Androgens |
Testosterone | Hypothyroidism | ||
Estradiol | Liver disease | ||
Thyroid-binding globulin | Thyroxine (T4) | Estrogen | Testosterone |
Triiodothyronine (T3) | Hyperthyroidism | Glucocorticoids | |
Liver disease | |||
Albumin | All lipid-soluble hormones | Estrogen | Liver disease |
Malnutrition | |||
Renal disease |
Hormone Receptors
When a hormone is released into the circulatory system, it is distributed throughout the body, but only those cells with appropriate hormone receptors for that hormone are affected. The target cell hormone receptors have two main functions: (1) to recognize and bind with high affinity to their particular hormones and (2) to initiate a signal to appropriate intracellular effectors. See Chapter 1 for cell signaling pathways, particularly Figures 1-19 and 1-20 on pp. 21-22.
The sensitivity of the target cell to a particular hormone is related to the total number of receptors per cell: the more receptors, the more sensitive the cell. Low concentrations of hormone increase the number of receptors per cell, called up-regulation (Figure 21-3, A). High concentrations of hormone decrease the number of receptors, called down-regulation (Figure 21-3, B). Thus the cell can adjust its sensitivity to the concentration of the signaling hormone. The receptors on the plasma membrane are continuously synthesized and degraded, so that changes in receptor concentration may occur within hours. Various physiochemical conditions also can affect both the receptor number and the affinity of the hormone for its receptor. Some of these physiochemical conditions are the fluidity and structure of the plasma membrane, pH, temperature, ion concentration, diet, and the presence of other chemicals (e.g., drugs). Finally, mutations in receptor structure can affect target cell activation such that normal cellular responses are increased or decreased. For example, mutations in thyroid hormone receptors can lead to resistance to thyroid hormone and can contribute to the development of tumors.1,2
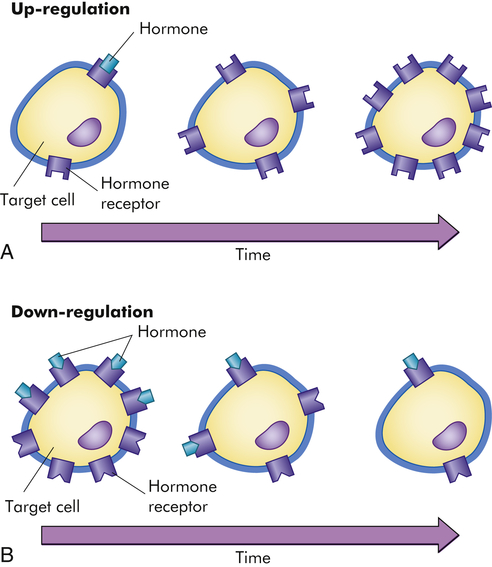
A, Low hormone level and up-regulation, or an increase in the number of receptors. B, High hormone level and down-regulation, or a decrease in the number of receptors. (From Patton KT, Thibodeau GA: Anatomy & physiology, ed 8, St Louis, 2013, Mosby.)
Hormone receptors may be located in or on the plasma membrane or in the intracellular compartment of the target cell (Figure 21-4). Water-soluble hormones (see Table 21-1) have a high molecular weight and cannot diffuse across the cell membrane. They interact or bind with receptors in or on the cell membrane and mediate short-acting responses.3 Lipid-soluble steroids, vitamin D, retinoic acid, and thyroid hormones diffuse freely across the plasma and nuclear membranes and bind with cytosolic or nuclear receptors (see Figure 21-4). The hormone-receptor complex binds to a specific region in the deoxyribonucleic acid (DNA) and stimulates the expression of a specific gene. Some lipid-soluble hormones (e.g., estrogen) also may bind with plasma membrane receptors. By these mechanisms, lipid-soluble hormones can mediate both long-acting and rapid-acting responses.4
Plasma Membrane Receptors and Signal Transduction
First Messenger
A hormone is the first messenger, is secreted into the bloodstream, and carries a message to a target cell. Signal transduction is the process by which this message is communicated into a cell. In general, signal transduction involves a series of steps that includes receptor activation or binding of a hormone to its receptor, activation of a G protein (transducer) and membrane-associated enzyme (effector enzyme), and production of a second messenger (see Figure 1-22, p. 24, and Figure 21-5). The final event is activation of an intracellular enzyme, such as protein kinase A or C, which causes alterations in gene transcription and the resulting target cell response to the hormone.
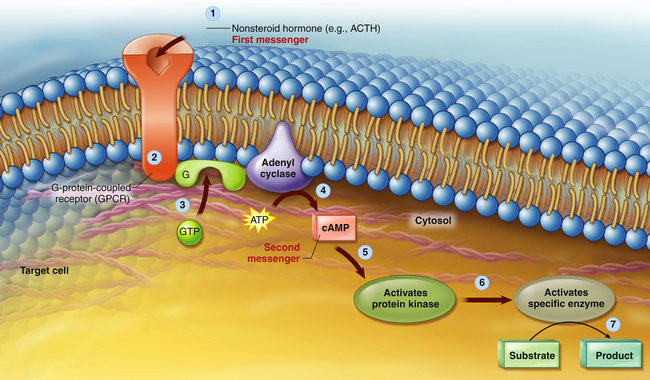
A nonsteroid hormone (first messenger) binds to a fixed receptor of the target cell (1). The hormone-receptor complex activates the G protein (2). The activated G protein (G) reacts with guanosine triphosphate (GTP), which in turn activates the membrane-bound enzyme adenylyl cyclase (3). Adenylyl cyclase catalyzes the conversion of adenosine triphosphate (ATP) to cyclic adenosine monophosphate (cAMP; second messenger) (4). cAMP activates protein kinase (5). Protein kinases activate specific intracellular enzymes (6). These activated enzymes then influence specific cellular reactions and metabolic pathways, thus producing the target cell’s response to the hormone (7). (From Patton KT, Thibodeau GA: Anatomy & physiology, ed 8, St Louis, 2013, Mosby). ACTH, Adrenocorticotropic hormone.
Second-Messenger Molecules: cAMP, cGMP, and Ca++
Cyclic Adenosine Monophosphate (cAMP)
Second-messenger molecules are the initial link between the first signal (hormone) and the inside of the cell (Table 21-3). For example, binding of epinephrine to a β-adrenergic receptor subtype activates (through a stimulatory G protein [Gs]) the enzyme adenylyl cyclase. Adenylyl cyclase catalyzes the conversion of adenosine triphosphate (ATP) to the second messenger 3′,5′-cAMP. Elevation of cAMP activates the enzyme cAMP-dependent protein kinase A (PKA). PKA phosphorylates and activates nuclear transcription factors (cAMP response element–binding [CREB] proteins) that influence numerous cellular functions.5 For example, CREB proteins associated with the L-type channel in cardiac muscle increase the influx of calcium into the cell, which increases myocardial contractility. Alterations in CREB activity have been implicated in many disease states including diabetes and cancer.6,7 The actions of cAMP are terminated by the enzyme phosphodiesterase (PDE) III, which hydrolyzes cAMP into inactive adenosine monophosphate (AMP).
TABLE 21-3
SECOND MESSENGERS IDENTIFIED FOR SPECIFIC HORMONES
SECOND MESSENGER | ASSOCIATED HORMONES |
Cyclic AMP | Adrenocorticotropic hormone (ACTH) |
Luteinizing hormone (LH) | |
Human chorionic gonadotropin (hCG) | |
Follicle-stimulating hormone (FSH) | |
Thyroid-stimulating hormone (TSH) | |
Antidiuretic hormone (ADH) | |
Thyrotropin-releasing hormone (TRH) | |
Parathyroid hormone (PTH) | |
Glucagon | |
Cyclic GMP | Atrial natriuretic peptide |
Calcium | Angiotensin II |
Gonadotropin-releasing hormone (GnRH) | |
Antidiuretic hormone (ADH) | |
IP3 and DAG | Angiotensin II |
Antidiuretic hormone (ADH) | |
Luteinizing hormone–releasing hormone (LHRH) | |
Tyrosine phosphorylation | |
Insulin | |
Growth hormone | |
Leptin | |
Prolactin |
Cyclic Guanosine Monophosphate (cGMP)
Guanylyl cyclase is an enzyme that converts guanosine triphosphate (GTP) to the second-messenger 3′,5′-cGMP. cGMP activates cGMP-dependent kinase (protein kinase G), which in turn activates a number of physiologic processes. The effects of various ligands, such as atrial natriuretic hormone (vascular smooth muscle relaxation) and nitric oxide (e.g., vascular smooth muscle relaxation and platelet inhibition), are mediated by the second-messenger cGMP. Drugs that target the actions of cGMP are being explored for the treatment of vascular and pulmonary disorders.8
Calcium (Ca++)
In addition to being an important ion that participates in a multitude of cellular actions, Ca++ is considered an important second messenger. The binding of a hormone (such as norepinephrine or angiotensin II) to a surface receptor activates the enzyme phospholipase C through a G protein inside the plasma membrane. This enzyme breaks down membrane phospholipid phosphatidylinositol biphosphate (PIP2) into second-messengers inositol triphosphate (IP3) and diacylglycerol (DAG) (see Figure 1-22, p. 24) IP3 mobilizes Ca++ from intracellular stores (endoplasmic reticulum). Increased intracellular calcium levels can lead to the formation of the calcium-calmodulin complex, which mediates the effects of calcium on intracellular activities that are crucial for cell metabolism and growth. For example, calmodulin-dependent protein kinases control intracellular contractile components (myosin and actin, which cause contraction), alter plasma membrane permeability to calcium, and regulate the intracellular enzyme activity that promotes hormone secretion.
DAG, together with Ca++, activates protein kinase C (PKC). Similar to other kinase enzymes, PKC activates (by phosphorylation) other proteins or enzymes. PKC initiates a variety of cellular responses that are linked to cell metabolism and growth. For example, PKC activates glycogen synthase in liver cells to convert glucose to glycogen. Calcium signaling systems are crucial to healthy functioning of virtually every tissue system in the body including heart, brain, bone, smooth muscle, and many others.9
Some hormones, such as insulin, growth hormone, and prolactin, bind to surface receptors that directly activate tyrosine kinases. These tyrosine kinases include the Janus family of tyrosine kinases (JAK) and signal transducers and activators of transcription (STAT). They regulate a wide range of intracellular processes that contribute to cellular metabolism and growth, and are being targeted in emerging treatments for diabetes.10 An example of first- and second-messenger systems is presented in Figure 21-5.
Steroid (Lipid-Soluble) Hormone Receptors
The lipid-soluble hormones are steroid hormones and are synthesized from cholesterol. They include androgens, estrogens, progestins, glucocorticoids, mineralocorticoids, vitamin D, and retinoid. Thyroid hormones are lipid soluble but are not synthesized from cholesterol (see p. 701). Because these hormones are relatively small, lipophilic, hydrophobic molecules, they can cross the lipid plasma membrane by simple diffusion (see Chapter 1). Some steroid hormones bind to receptor molecules in the cytoplasm and then diffuse into the nucleus, whereas others bind to receptors in the nucleus. The resulting hormone-receptor complex binds to a specific site on the promoter region of DNA. This binding activates ribonucleic acid (RNA) polymerase, which stimulates DNA transcription and increased synthesis of specific proteins (increased gene expression) (Figure 21-6). Modulation of gene expression can take hours to days.
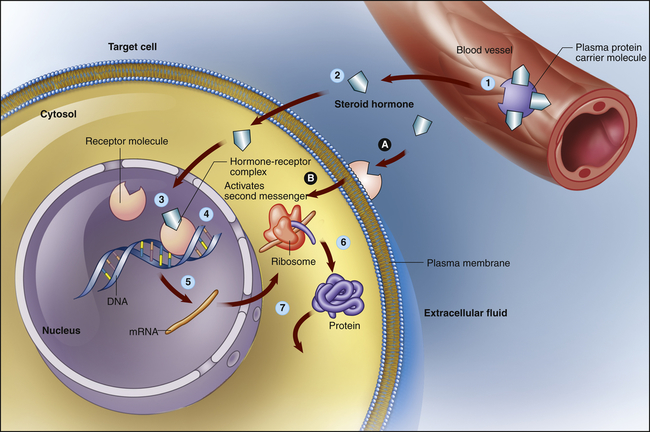
Lipid-soluble steroid hormone molecules detach from the carrier protein (1) and pass through the plasma membrane (2). Hormone molecules then diffuse into the nucleus, where they bind to a receptor to form a hormone-receptor complex (3). This complex then binds to a specific site on a DNA molecule (4), triggering transcription of the genetic information encoded there (5). The resulting messenger ribonucleic acid (mRNA) molecule moves to the cytosol, where it associates with a ribosome, initiating synthesis of a new protein (6). This new protein—usually an enzyme or channel protein—produces specific effects on the target cell (7). The classic genomic action is typically slow (red arrows). Steroids also may exact rapid effects by binding to receptors on the plasma membrane (A) and activating an intercellular second messenger (B). (Modified from Patton KT, Thibodeau GA: Anatomy & physiology, ed 8, St Louis, 2013, Mosby.)
Steroid hormone receptors also may be found in the plasma membrane and are associated with rapid responses (seconds or minutes) that have nongenomic and genomic effects. Crosstalk between gene transcription and nongenomic responses modulate each other, allowing cells to adapt rapidly to environmental changes. Thyroid hormone, a nonsteroid lipid-soluble hormone, also has been found to use a cell surface receptor for its nongenomic actions. It first binds to an integrin receptor on the plasma membrane, and then uses a specific transport mechanism to gain access to its nuclear receptors where it can influence cell division and metabolic function.11
Structure and Function of the Endocrine Glands
Hypothalamic-Pituitary Axis
The hypothalamic-pituitary axis (HPA) forms the structural and functional basis for central integration of the neurologic and endocrine systems, creating what is called the neuroendocrine system. The HPA produces a number of releasing/inhibitory hormones and tropic hormones that affect a number of diverse body functions (Figure 21-7). For example, the functions of the thyroid gland, adrenal gland, and male and female reproductive glands, as well as somatic growth and lactation, are regulated by hormones originating from the HPA.
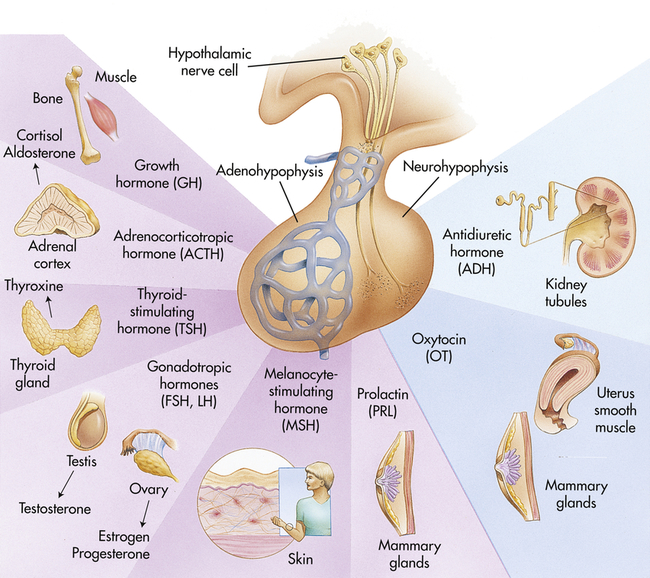
FSH, Follicle-stimulating hormone; LH, luteinizing hormone. (Modified from Patton KT, Thibodeau GA: Anatomy & physiology, ed 8, St Louis, 2013, Mosby.)
Hypothalamus
The hypothalamus is divided into several nuclei and nuclear areas and is located at the base of the brain. The pituitary gland is located at the sella turcica, a saddle-shaped depression on the superior surface of the sphenoid bone (Figure 21-8). The communication or anatomic connection (blood vessels and neural tract) between the hypothalamus and anterior and posterior pituitary is quite elaborate and well described. However, simply described, the hypothalamus is connected to the anterior pituitary by way of portal blood vessels (Figure 21-9), whereas the hypothalamus is connected to the posterior pituitary by way of a nerve tract referred to as the supraopticohypophysial tract (Figure 21-10). These connections are vital to the functioning of the hypothalamus-pituitary system.12
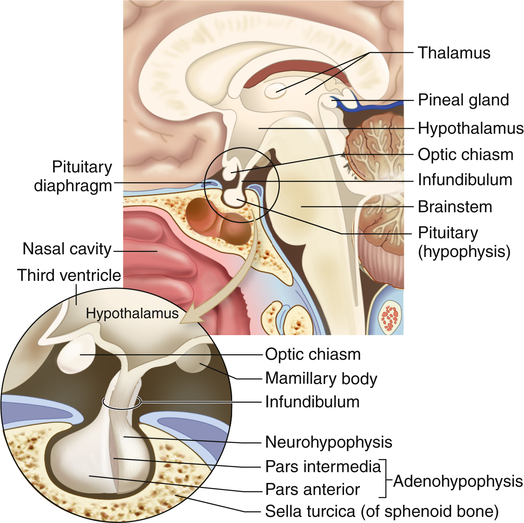
The pituitary gland is located within the sella turcica of the skull’s sphenoid bone and is connected to the hypothalamus by a stalklike infundibulum. The infundibulum passes through a gap in the portion of the dura mater that covers the pituitary (the pituitary diaphragm). The inset shows that the pituitary is divided into an anterior portion, the adenohypophysis, and a posterior portion, the neurohypophysis. The adenohypophysis is further subdivided into the pars anterior and pars intermedia. The pars intermedia is almost absent in the adult pituitary. (Modified from Patton KT, Thibodeau GA: Anatomy & physiology, ed 8, St Louis, 2013, Mosby.)
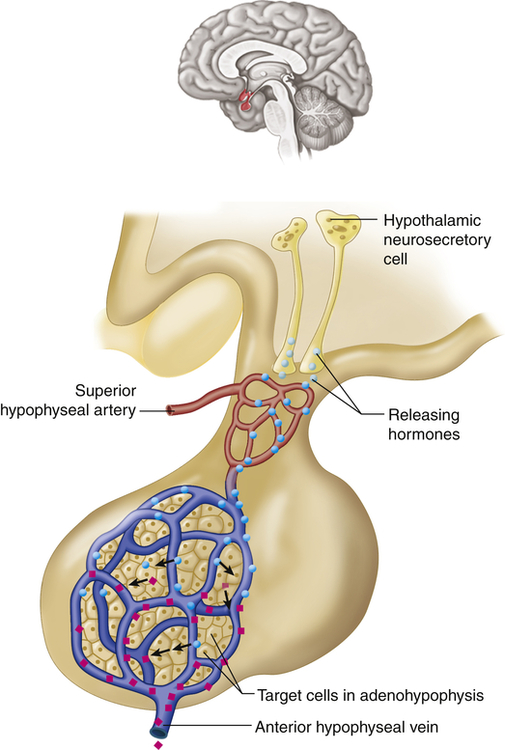
Neurons in the hypothalamus secrete releasing hormones into veins that carry the releasing hormones directly to the vessels of the adenohypophysis, thus bypassing the normal circulatory route. (From Patton KT, Thibodeau GA: Anatomy & physiology, ed 8, St Louis, 2013, Mosby.)
The special cells of the hypothalamus are like other neurons in that they have similar electrical properties, organelles, membranes, and synapses. Hypothalamic neurosecretory cells, however, can synthesize and secrete the hypothalamic-releasing hormones and synthesize the hormones of the posterior portion of the pituitary gland. For example, antidiuretic hormone (ADH) and oxytocin are synthesized in hypothalamic neurons but are stored and secreted by the posterior pituitary. ADH and oxytocin travel to the posterior pituitary by way of the hypothalamohypophysial nerve tract. Releasing/inhibitory hormones also are synthesized in the hypothalamus and are secreted into the portal blood vessels, through which they travel to the anterior pituitary and control the release of tropic hormones. These releasing/inhibitory hormones from the hypothalamus include prolactin-inhibiting factor (PIF), thyrotropin-releasing hormone (TRH), gonadotropin-releasing hormone (GnRH), somatostatin, growth hormone–releasing factor (GRF), corticotropin-releasing hormone (CRH), and substance P. These hormones are summarized in Table 21-4.
TABLE 21-4
HYPOTHALAMIC HORMONES (HYPOPHYSIOTROPIC HORMONES)
HORMONE | TARGET TISSUE | ACTION |
Thyrotropin-releasing hormone (TRH) | Anterior pituitary | Stimulates release of thyroid-stimulating hormone (TSH) Modulates prolactin secretion |
Gonadotropin-releasing hormone (GnRH) | Anterior pituitary | Stimulates release of follicle-stimulating hormone (FSH) and luteinizing hormone (LH) |
Somatostatin | Anterior pituitary | Inhibits release of growth hormone (GH) and TSH |
Growth hormone–releasing hormone (GHRH) | Anterior pituitary | Stimulates release of GH |
Corticotropin-releasing hormone (CRH) | Anterior pituitary | Stimulates release of adrenocorticotropic hormone (ACTH) and β-endorphin |
Substance P | Anterior pituitary | Inhibits synthesis and release of ACTH Stimulates secretion of GH, FSH, LH, and prolactin |
Dopamine | Anterior pituitary | Inhibits synthesis and secretion of prolactin |
Prolactin-releasing factor (PRF) | Anterior pituitary | Stimulates secretion of prolactin |
Pituitary Gland
Because of the anatomic location and connection of the pituitary gland to the brain, several neurotransmitters as well as physical and emotional stressors influence the release of specific hypothalamic releasing–inhibitory hormones and their respective tropic hormones. This allows for the integrated and coordinated function of the hypothalamic-pituitary axis. Interestingly, hypothalamic hormones also are synthesized outside the HPA. For example, CRH is synthesized in cells of the immune system, female and male reproductive organs, and the placenta. These peripherally synthesized neuropeptides are thought to play a role in the reproductive and immune responses to stress.13,14
Hormones of the Posterior Pituitary
The posterior pituitary secretes two polypeptide hormones: (1) ADH, also called arginine-vasopressin; and (2) oxytocin. These peptide hormones are similar in structure, differing by only two amino acids. They are synthesized, along with their carrier proteins (the neurophysins), in the supraoptic and paraventricular nuclei of the hypothalamus (see Figure 21-10). Once synthesized, these hormones and their neurophysins are packaged in secretory vesicles and are moved down the axons of the pituitary stalk to the pars nervosa for storage. The posterior pituitary thus can be seen as a storage and releasing site for hormones synthesized in the hypothalamus.
The release of ADH and oxytocin is mediated by cholinergic and adrenergic neurotransmitters. The major stimulus to both ADH and oxytocin release is glutamate, whereas the major inhibitory input is through gamma-aminobutyric acid (GABA). Before release into the circulatory system, ADH and oxytocin are split from the neurophysins and are secreted in unbound form.15
Antidiuretic Hormone
The major homeostatic function of the posterior pituitary is the control of plasma osmolality, as regulated by ADH (see Chapter 3). At physiologic levels, ADH acts on the vasopressin 2 (V2) receptors of the renal tubular cells to increase their permeability (see Chapter 37). This increased permeability leads to an increase in water reabsorption into the blood and the production of more concentrated urine. These effects may be inhibited by hypercalcemia, prostaglandin E, and hypokalemia. At pathophysiologically high serum levels, ADH acts on vasopressin 1 (V1) receptors and causes vasoconstriction.
The secretion of ADH is regulated primarily by the osmoreceptors of the hypothalamus, located near or in the supraoptic nuclei. As plasma osmolality increases, these osmoreceptors are stimulated, the rate of ADH secretion increases, more water is reabsorbed from the kidney, and the plasma is diluted to its set-point osmolality (approximately 280 mOsm/kg).15 ADH has no direct effect on electrolyte levels, but by increasing water reabsorption, serum electrolyte concentrations may decrease because of a dilutional effect.
As mentioned previously, ADH at high serum levels acts on the V1 receptors and causes vasoconstriction and a resulting increase in arterial blood pressure. This baroreceptor-mediated response is much less sensitive than the ADH response to changes in osmolarity. Therefore, physiologic levels of ADH do not significantly affect vessel tone. However, significant vasoconstriction may be achieved pharmacologically. For example, high doses of ADH (given as the drug vasopressin) may be administered to achieve hemostasis during hemorrhage and to raise blood pressure in shock states.16
Oxytocin
Oxytocin is responsible for contraction of the uterus and milk ejection in lactating women and may affect sperm motility in men. In a woman, oxytocin is secreted in response to suckling and mechanical distention of the female reproductive tract. Stimulated by sucking, oxytocin binds to its receptors on myoepithelial cells in the mammary tissues and causes contraction of those cells. This results in increased intramammary pressure and milk expression (“let down” reflex). In response to distention of the uterus, oxytocin stimulates contractions. Oxytocin functions near the end of labor to enhance the effectiveness of contractions, promote delivery of the placenta, and stimulate postpartum uterine contractions, thereby preventing excessive bleeding. The function of this hormone is discussed in more detail in Chapter 23.
Oxytocin has been implicated in behavior responses, especially in women. It has been suggested that oxytocin and its receptor play a role in the brain’s responsiveness to stressful stimuli, especially in the pregnant and postpartum states. Its potential role in the treatment of maternal child neglect and a variety of anxiety disorders is being explored.17,18
Hormones of the Anterior Pituitary
The anterior pituitary is composed of two main cell types: (1) the chromophobes, which appear to be nonsecretory; and (2) the chromophils, which are considered the secretory cells of the adenohypophysis. The chromophils are subdivided into seven secretory cell types, each type secreting one or more specific hormones (Table 21-5). In general, the regulation of the anterior pituitary hormones is achieved by (1) feedback of hypothalamic releasing–inhibitory hormones and factors, (2) feedback from target gland hormones (i.e., cortisol, estrogen), and (3) direct effects of neurotransmitters.
TABLE 21-5
TROPIC HORMONES OF THE ANTERIOR PITUITARY AND THEIR FUNCTIONS
HORMONE | SECRETORY CELL TYPE | TARGET ORGANS | FUNCTIONS |
Adrenocorticotropic hormone (ACTH) | Corticotropic | Adrenal gland (cortex) | Increased steroidogenesis (cortisol and androgenic hormones) Synthesis of adrenal proteins contributing to maintenance of the adrenal gland |
Melanocyte-stimulating hormone (MSH) | Melanotropic | Anterior pituitary | Promotes secretion of melanin and lipotropin by anterior pituitary; makes skin darker |
Somatotropic hormones | |||
Somatotropic | Muscle, bone, liver | Regulates metabolic processes related to growth and adaptation to physical and emotional stressors, muscle growth, increased protein synthesis, increased liver glycogenolysis, increased fat mobilization | |
Liver | Induces formation of somatomedins, or insulin-like growth factors (IGFs) that have actions similar to insulin | ||
Lactotropic | Breast | Milk production | |
Glycoprotein hormones | |||
Thyrotropic | Thyroid gland | Increased production and secretion of thyroid hormone Increased iodide uptake Promotes hypertrophy and hyperplasia of thymocytes | |
Get Clinical Tree app for offline access ![]() |