1 Introduction
Laboratory evaluation of antimicrobial agents remains a cornerstone of clinical microbiology and antimicrobial/ biocide discovery and development. The development of robust and reproducible assays for determining microbial susceptibility to antimicrobial agents is of fundamental importance in the appropriate selection of therapeutic agents and biocides for use in infection control, disinfection, preservation and antifouling applications. Such laboratory assays form the basis for high-throughput screening of compounds or biological extracts in the discovery, isolation and development of new antimicrobial drugs and biocides. Such assays facilitate identification of antimicrobial agents from various sources and in lead antimicrobial compound optimization. In the control of human and animal infection, laboratory evaluation of candidate agents yields crucial information which can inform choice of antimicrobial agent(s) where the causative organism is known or suspected. As the number of microorganisms exhibiting resistance to conventional antimicrobial agents increases, laboratory evaluation of antimicrobial susceptibility is increasingly important for the selection of appropriate therapeutic antimicrobials. Evaluation of the potential antimicrobial action and nature of the inhibitory or lethal effects of established and novel therapeutic agents and biocides are important considerations in the success of therapeutic interventions and infection/contamination control procedures.
Significant concerns that the extensive use of biocidal agents may be linked to the development of antimicrobial resistance exist. Recent concerns regarding significant global public health issues such as the increasing threat of bioterrorism, the prevalence of healthcare associated infections, severe acute respiratory syndrome (SARS), avian influenza (H5N1) and the 2009 World Health Organization declaration of the swine flu (H1N1) pandemic (the first pandemic of the 21st century) have seen global demand for biocides increase dramatically. In addition, the emergence of new infectious agents (e.g. prions) and the increasing transmission rates of significant blood-borne viruses (e.g. HIV, hepatitis B and C) which may readily contaminate medical instruments or the environment has focused attention on the need for effective and proven disinfecting and sterilizing agents.
Finally, increasing appreciation of the role played by microbial biofilms in human and animal infectious diseases and their ubiquitous distribution in natural ecosystems has led to the development of novel approaches for the laboratory evaluation of antimicrobial susceptibility of microorganisms growing as surface-adhered sessile populations. These studies have demonstrated that microorganisms in the biofilm mode of growth are phenotypically different from their planktonic counterparts and frequently exhibit significant tolerance to antimicrobial challenge (see Chapter 8). This has implications for the environmental control of microorganisms and in the selection of appropriate concentrations of antibiotic or biocide necessary to eradicate them. As such, biofilms may constitute a reservoir of infectious microorganisms which may remain following antimicrobial treatment, even if antimicrobial selection is based on standard laboratory evaluations of antimicrobials which are based on planktonic cultures of microorganisms. Tests for evaluating candidate antimicrobial agents to be used in human and animal medicine as well as environmental biocides remain significant laboratory considerations.
1.1 Definitions
Key terms such as disinfection, preservation, antisepsis and sterilization are defined in Chapters 19 and 21. A number of other important terms used to describe the antimicrobial activity of agents are also commonly used. A biocide may be defined as a chemical or physical agent which kills viable organisms, both pathogenic and nonpathogenic. This broad definition clearly includes microoganisms, but is not restricted to them. The term microbicide is therefore also used to refer specifically to an agent which kills microorganisms (germicide may also be used in this context, but generally refers to pathogenic microorganisms). The terms biocidal, bactericidal, fungicidal and viricidal therefore describe an agent with killing activity against a specific class or classes of organism indicated by the prefix, whereas the terms bacteriostatic and fungistatic refer to agents which inhibit the growth of bacteria or fungi (Figure 18.1), but do not necessarily kill them. It should be noted, however, that some microorganisms that appear non-viable and non-cultivable fol-lowing antimicrobial challenge may be revived by appropriate methods, and that organisms incapable of multiplication may retain some enzymatic activity.
Figure 18.1 Effect on the subsequent growth pattern of inhibitory (static,Δ) or cidal () agents added at time X (the normal growth pattern is indicated by the · line).
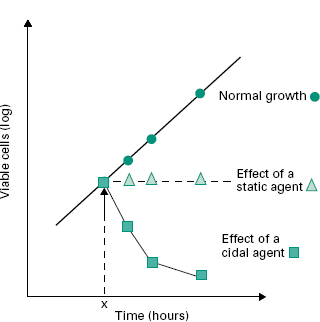
In the laboratory evaluation of antibacterial agents, the terms minimum inhibitory concentration (MIC) and minimum bactericidal concentration (MBC) are most commonly used. Recently published British Society for Chemotherapy (BSAC) guidelines for the determination of minimum inhibitory concentrations (see Further Reading) define the MIC as the lowest concentration of antimicrobial which will inhibit the visible growth of a microorganism after overnight cultivation and the MBC as the lowest concentration of antimicrobial that will prevent the growth of a microorganism after subculture onto antibiotic-free media. Generally, MIC and MBC values are recorded in milligrams per litre or per millilitre (mg/L or mg/ml). With most cidal antimicrobials, the MIC and MBC are frequently near or equal in value, although with essentially static agents (e.g. tetracycline), the lowest concentration required to kill the microorganism(i.e. the MBC) is invariably many times the MIC and often clinically unachievable without damage to the human host. As with microbicides, cidal terms can be applied to studies involving not just bacteria but other microbes, e.g. when referring to cidal antifungal agents the term minimum fungicidal concentration (MFC) is used. Recently, thanks to developments in the design of high-throughput laboratory screens for biofilm suscepti-bility, the minimum biofilm eradication concentration (MBEC) can be accurately determined for organisms grown as single or mixed species biofilms. The MBEC is the minimum concentration of an antimicrobial agent required to kill a microbial biofilm. For conventional antibiotics and biocides the MBEC value may be 1000-fold higher than the MBC value for the same planktonic microorganisms. Further studies have shown that often no correlation exists between the MIC and the MBEC, indicating the potential limitations of therapeutic antibiotic selection based on determined MIC values.
The term tolerance implies the ability of some bacterial strains to survive (without using or expressing resistance mechanisms), but not grow, at levels of antimicrobial agent that should normally be cidal. This applies particularly to systems employing the cell-wall-active β-lactams and glycopeptides, and to Gram-positive bacteria such as streptococci. Normally, MIC and MBC levels in such tests should be similar (i.e. within one or two doubling dilu-tions); if the MIC/MBC ratio is 32 or greater, the term tolerance is used. Tolerance may in some way be related to the Eagle phenomenon (paradoxical effect), where increasing concentrations of antimicrobial result in reduced killing rather than the increase in cidal activity expected (see Figure 18.2). Tolerance to elevated antimicrobial challenge concentrations is also a characteristic of microbial biofilm populations. Finally, the term resistance has several definitions within the literature, however, it generally refers to the ability of a microorganism to withstand the effects of a harmful chemical agent, with the organism neither killed nor inhibited at concentrations to which the majority of strains of that organism are susceptible. Resistance mechanisms generally involve modification of the normal target of the antimicrobial agent either by mutation, enzymatic changes, target substitution, antibiotic destruction or alteration, antibiotic efflux mechanisms and restricted permeability to antibiotics.
Figure 18.2 Survival of Enterococcus faecalis exposed to a fluoroquinolone for 4 hours at 37°C. Three initial bacterial concentrations were studied, 107 Cfu/ml (); 106 CFU/ml (Δ) and 105 CFU/ml (
). This clearly demonstrates a paradoxical effect (increasing antimicrobial concentrations past a critical level reveal decreased killing), and the effects of increased inoculum densities on subsequent killing. (Courtesy of Dr Z. Hashmi.)
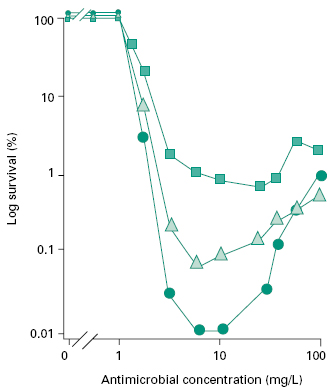
2 Factors affecting the antimicrobial activity of disinfectants
The activity of antimicrobial agents on a given organism or population of organisms will depend on a number of factors which must be reflected in the tests used to define their efficacy. For example, the activity of a given antimicrobial agent will be affected by nature of the agent, the nature of the challenge organism, the mode of growth of the challenge organism, concentration of agent, size of the challenge population and duration of exposure environmental/physical conditions (temperature, pH, presence of extraneous organic matter) are also important considerations in modelling the activity of biocidal agents. Laboratory tests for the evaluation of biocidal activity must be carefully designed to take into account these factors which may significantly influence the rate of kill within the microbial challenge population.
The work of Krönig and Paul in the late 1890s, demonstrated that the rate of chemical disinfection was related to both concentration of the chemical agent and the temperature of the system, and that bacteria exposed to a cidal agent do not die simultaneously but in an orderly sequence. This led to various attempts at applying the kinetics of pure chemical reactions (the mechanistic hypothesis of disinfection) to microbe/disinfectant inter-actions. However, since the inactivation kinetics depend on a large number of defined and undefined variables, such models are often too complicated for routine use. Despite this, the Chick-Watson model (equation 1), based on first-order reaction kinetics, remains the basic rate law for the examination of disinfection kinetics:
(1)
where N is the number of surviving microbes after time t and k0 is the disinfection rate constant. The Chick-Watson model may be further refined to account for biocide concentration (equation 2):
(2)
where k1 is the concentration-independent rate constant, B is the biocide concentration and n is the dilution coef-ficient. The Chick-Watson model predicts that the number of survivors falls exponentially at a rate governed by the rate constant and the concentration of disinfect-ant. A general assumption is that the concentration of biocide remains constant throughout the experiment; however, there are a number of situations when this appears not to be the case (sequestering) and may result in observed departures from linear reaction kinetics. The factors influencing the antimicrobial activity of disinfectant agents are discussed below.
2.1 Innate (natural) resistance of microorganisms
The susceptibility of microorganisms to chemical disinfectants and biocides exhibits tremendous variation across various classes and species. Bacterial endospores and the mycobacteria (e.g. Mycobacterium tuberculosis) possess the most innate resistance, while many vegetative bacteria and some viruses appear highly susceptible (see Chapter 19). In addition, microorganisms adhering to surfaces as biofilms or present within other cells (e.g. legionellae within amoebae), may reveal a marked increase in resistance to disinfectants and biocides (Figure 18.3). Therefore, when evaluating new disinfectants, a suitable range of microorganisms and environmental conditions must be included in tests. The European suspension test (EN 12054) for hospital-related studies and the European/British Standard suspension test (EN 1276) for studies relating to food, industrial, institutional and domestic areas, both include Pseudomonas aeruginosa, Escherichia coli, Staphylococcus aureus and Enterococcus hirae as the challenge organisms to be used in the test. For specific applications, additional strains may be chosen from Salmonella enterica serovar Typhimurium, Lactobacillus brevis and Enterobacter cloacae.
Figure 18.3 Survival of stationary phase broth cultures of Legionella pneumophila and amoebae-grown L. pneumophila after exposure to 32 mg/L benzisothiazolone (Proxel) at 35°C in Ringer’ s solution. (Adapted from Barker et al. (1992), Appl Environ Microbiol, 58, 2420–2425, with permission.)
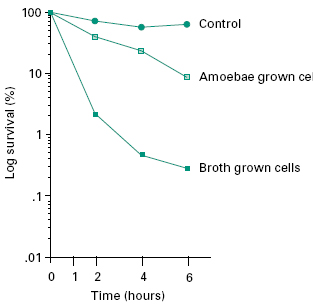
2.2 Microbial density
Many disinfectants require adsorption to the microbial cell surface prior to killing, therefore dense cell populations or sessile populations may sequester all the available disinfectant before all cells are affected, thus shielding a proportion of the population from the toxic effects of the chemical agent. Therefore, from a practical point of view, the larger the number of microorganisms present, the longer it takes a disinfectant to complete killing of all cells. For instance, using identical test conditions, it has been shown that 10 spores of the anthrax bacillus (Bacillus anthracis) were destroyed in 30 minutes, while it took 3 hours to kill 100 000 (105) spores. The implications of predisinfection washing and cleaning of objects (which removes most of the microorganisms) becomes obvious. However, when evaluating disinfectants in the laboratory, it must be remembered that unlike sterilization, kill curves with disinfectants may not be linear and the rate of killing may decrease at lower cell numbers (Figure 18.3). Hence a 3-log killing may be more rapidly achieved with 108 than 104 cells. Johnston et al. (2000) demonstrated that even small variations in the initial inoculum size (Staph. η= aureus) had a dramatic effect on log reductions over time, using a constant concentration of sodium dodecyl sulphate (SDS). The authors argue that the presence of microbes quenches the action of the biocide (selfquenching), since cell and membrane components of lysed bacteria (e.g. emulsifiers such as triacylglycerols and phosphatidyl ethanolamine), are similar in action to emulsifiers (such as Tween and lecithin) used in standard biocide quenching/neutralizing agents employed in dis-infectant tests. However, this may not hold true across all biocides where similar inoculum size dependency of disinfection is observed (see Russell et al., 1997). Initial bioburden/cell numbers must, therefore, be standardized and accurately quantified in disinfectant efficacy (suspen-sion) tests and agreement reached on the degree of killing required over a stipulated time interval (see Table 18.1).
Table 18.1 Methods of recording viable cells remaining after exposure of an initial population of 1 000 000 (106) CFU to a cidal agent
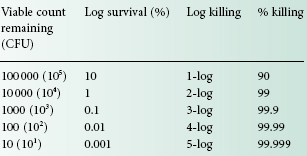
2.3 Disinfectant concentration and exposure time
The effects of concentration or dilution of the active ingredient on the activity of a disinfectant are of major importance. With the exception of iodophors, the more concentrated a disinfectant, the greater its efficacy, and the shorter the time of exposure required to destroy the population of microorganisms, i.e. there is an exponential relationship between potency and concentration. Therefore, a graph plotting the log10 of the death time (i.e. the time required to kill a standard inoculum) against the log10 of the concentration is typically a straight line, the slope of which is the concentration exponent (η). Expressed as an equation:
(3)
Thus η can be obtained from experimental data either graphically or by substitution in equation (3) (see Table 18.2).
Table 18.2 Concentration exponents, η, for some disinfectant substances
QAC, quaternary ammonium compound.
Antimicrobial agent | η |
Hydrogen peroxide | 0.5 |
Silver nitrate | 0.9–1.0 |
Mercurials | 0.03–3.0 |
Iodine | 0.9 |
Crystal violet | 0.9 |
Chlorhexidine | 2 |
Formaldehyde | 1 |
QACs | 0.8–2.5 |
Acridines | 0.7–1.9 |
Formaldehyde donors | 0.8–0.9 |
Bronopol | 0.7 |
Polymeric biguanides | 1.5–1.6 |
Parabens | 2.5 |
Sorbic acid | 2.6–3.2 |
Potassium laurate | 2.3 |
Benzyl alcohol | 2.6–4.6 |
Aliphatic alcohols | 6.0–12.7 |
Glycolmonophenyl ethers | 5.8–6.4 |
Glycolmonoalkyl ethers | 6.4–15.9 |
Phenolic agents | 4.0–9.9 |
It is important to note that dilution does not affect the cidal attributes of all disinfectants in a similar manner. For example, mercuric chloride with a concentration exponent of 1 will be reduced by the power of 1 on dilu-tion, and a threefold dilution means the disinfectant activity will be reduced by the value 31, i.e. to a third of its original activity. Phenol, however, has a concentration exponent of 6, so a threefold dilution in this case will mean a decrease in activity of 36 or 729 times less active than the original concentration. Thus, the likely dilution experienced by the disinfectant agent in use must be given due consideration when selecting an appropriate biocidal agent for a given application.
2.4 Physical and chemical factors
Known and proven influences include temperature, pH and mineral content of water (‘hardness’).
2.4.1 Temperature
As with most chemical/biochemical reactions, the cidal activity of most disinfectants increases with increase in temperature, since temperature is a measure of the kinetic energy within a reaction system. Increasing the kinetic energy of a reaction system increases the rate of reaction by increasing the number of collisions between reactants per unit time. This process is observed up to an optimum temperature, beyond which reaction rates fall again, due to thermal denaturation of some component(s) of the reaction. As the temperature is increased in arithmetical progression the rate (velocity) of disinfection increases in geometrical progression. Results may be expressed quantitatively by means of a temperature coefficient, either the temperature coefficient per degree rise in temperature (θ), or the coefficient per 10°C rise (the Q10 value) (Hugo & Russell, 1998). As shown by Koch, working with phenol and anthrax (B. anthracis) spores over 120 years ago, raising the temperature of phenol from 20°C to 30°C increased the killing activity by a factor of 4 (the Q10 value).
The value of θ may be calculated from the equation:
(4)
where t1 is the extinction time at T1°C, and t2 the extinction at T2°C (i.e. T1 + 1°C).
Q10 values may be calculated easily by determining the extinction time at two temperatures differing by exactly 10°C. Then:
(5)
It is also possible to plot the rate of kill against the temperature.
While the value for Q10 of chemical and enzyme-catalysed reactions lies in a narrow range (between 2 and 3), values for disinfectants vary widely, e.g. 4 for phenol, 45 for ethanol, and almost 300 for ethylene glycol monoethyl ether. Clearly, relating chemical reaction kinetics to disinfection processes is potentially dangerous. Most laboratory tests involving disinfectant-like chemicals are now standardized to 20°C, i.e. around ambient room temperatures.
2.4.2 pH
Effects of pH on antimicrobial activity can be complex. As well as directly influencing the survival and rate of growth of the microorganism under test, changes in pH may affect the potency of the agent and its ability to interact with cell surface sites. In a many cases (where the biocidal agent is an acid or a base), the ionization state (or degree of ionization) will depend on the pH. As is the case with some antimicrobials (e.g. phenols, acetic acid, benzoic acid), the non-ionized molecule is the active state (capable of crossing the cell membrane/partitioning) and alkaline pHs which favour the formation of ions of such compounds will decrease the activity. For these biocidal agents a knowledge of the molecule’s p Ka is important in predicting the pH range over which activity can be observed, since in situations where the pH of the system equals the p Ka of the biocide molecule, ionized and unionized species are in equilibrium. Others, such as glutaraldehyde and quaternary ammonium compounds (QACs), reveal increased cidal activity as the pH rises and are best used under alkaline conditions, possibly due to enhanced interaction with amino groups on microbial biomolecules. The pH also influences the properties of the bacterial cell surface, by increasing the proportion of anionic groups and hence its interaction with cidal molecules. Since the activity of many disinfectants requires adherence to cell surfaces; increasing the external pH renders cell surfaces more negatively charged and enhances the binding of cationic compounds such as chlorhexidine and QACs.
2.4.3 Divalent cations
The presence of divalent cations (e.g. Mg2+, Ca2+), for example in hard water, has been shown to exert an antagonistic effect on certain biocides while having an additive effect on the cidal activity of others. Metal ions such as Mg 2+ and Ca2+ may interact with the disinfectant itself to form insoluble precipitates and also interact with the microbial cell surface and block disinfectant adsorption sites necessary for activity. Biguanides, such as chlorhexi-dine, are inactivated by hard water. Hard water should always be employed for laboratory disinfectant and antiseptic evaluations to reflect this, with recommended formulae employing various concentrations of MgCl2 and CaCl2 solutions, available from the World Health Organization and the British Standard (BS EN 1276). On the other hand, cationic compounds may disrupt the outer membrane of Gram-negative bacteria and facilitate their own entry.
2.5 Presence of extraneous organic material
The presence of extraneous organic material such as blood, serum, pus, faeces or soil is known to affect the cidal activity of many antimicrobial agents. Therefore, it is necessary to determine the likely interaction between organic matter and the disinfectant by including this parameter in laboratory evaluations of their activity. In order to simulate ‘clean’ conditions (i.e. conditions of minimal organic contaminant), disinfectants are tested in hard water containing 0.3 g/L bovine albumin, with the albumin being used to mimic ‘dirty’ conditions. This standardized method replaces earlier approaches, some of which employed dried human faeces or yeast to mimic the effects of blood, pus or faeces on disinfectant activity. Disinfectants whose activities are particularly attenuated in the presence of organic contaminant include the halogen disinfectants (e.g. sodium hypochlo-rite) where the disinfectant reacts with the organic matter to form inactive complexes, biguanides, phenolic compounds and QACs. The aldehydes (formaldehyde and glutaraldehyde) are largely unaffected by the presence of extraneous organic contaminants. Organic material may also interfere with cidal activity by adherence to the microbial cell surface and blockade of adsorption sites necessary for disinfectant activity. For practical purposes and to mirror potential in-use situations, disinfectants should be evaluated under both clean and dirty conditions. Alternatives to albumin have also been suggested, for example sheep blood or mucin.
3 Evaluation of liquid disinfectants
3.1 General
Phenol coefficient tests were developed in the early 20th century when typhoid fever was a significant public health problem and phenolics were used to disinfect contaminated utensils and other inanimate objects. Details of such tests can be found in earlier editions of this book. However, as non-phenolic disinfectants became more widely available, tests that more closely paralleled the conditions under which disinfectants were being used (e.g. blood spills) and which included a more diverse range of microbial types (e.g. viruses, bacteria, fungi, protozoa) were developed. Evaluation of a disin-fectant’s efficacy was based on its ability to kill microbes, i.e. its cidal activity, under environmental conditions mimicking as closely as possible real life situations. As an essential component of each test was a final viability assay, removal or neutralization of any residual disinfectant (to prevent ‘carryover’ toxicity) became a significant consideration.
The development of methods to evaluate disinfectant activity in diverse environmental conditions and to determine suitable in-use concentrations/dilutions to be used led to the development by Kelsey, Sykes and Maurer of the so-called capacity-use dilution test which measured the ability of a disinfectant at appropriate concentrations to kill successive additions of a bacterial culture. Results were reported simply as pass or fail and not a numerical coefficient. Tests employed disinfectants diluted in hard water (clean conditions) and in hard water containing organic material (yeast suspension to simulate dirty con-ditions), with the final recovery broth containing 3% Tween 80 as a neutralizer. Such tests are applicable for use with a wide variety of disinfectants (see Kelsey & Maurer, 1974). Capacity tests mimic the practical situations of housekeeping and instrument disinfection, where surfaces are contaminated, exposed to disinfectant, recontaminated and so forth. The British Standard (BS 6907:1987) method for estimation of disinfectants used in dirty conditions in hospitals by a modification of the original Kelsey-Sykes test is the most widely employed capacity test in the UK and Europe. In the USA, effectiveness test data for submission must be obtained by methods accepted by the Association of Official Analytical Chemists, known collectively as Disinfectant Effectiveness Tests (DETs).
However, the best information concerning the fate of microbes exposed to a disinfectant is obtainable by counting the number of viable cells remaining after exposure of a standard suspension of cells to the disinfectant at known concentration for a given time interval— suspension tests. Viable counting is a facile technique used in many branches of pure and applied microbiology. Assessment of the number of viable microbes remaining (survivors) after exposure allows the killing or cidal activity of the disinfectant to be expressed in a variety of ways, e.g. percentage kill (e.g. 99.999%), as a log10 reduction in numbers (e.g. 5-log killing), or by log10 survival expressed as a percentage. Examples of such outcomes are shown in Table 18.1.
Unfortunately, standardization of the methodology to be employed in these efficacy tests has proven difficult, if not impossible, to obtain, as has consensus on what level of killing represents a satisfactory and/or acceptable result. It must be stressed, however, that unlike tests involving chemotherapeutic agents where the major aim is to establish antimicrobial concentrations that inhibit growth (i.e. MICs), disinfectant tests require determinations of appropriate cidal levels. Levels of killing required over a given time interval tend to vary depending on the regulatory authority concerned. While a 5-log killing of bacteria (starting with 106 CFU/ml) has been suggested for suspension tests, some authorities require a 6-log killing in simulated use tests. With viruses, a 4-log killing tends to be an acceptable result, while with prions it has been recommended that a titre loss of 104 prions should be regarded as an indication of appropriate disinfection provided that there has been adequate prior cleaning. With simulated use tests, cleaning followed by appropriate disinfection should result in a prion titre loss of at least 107.
3.2 Antibacterial disinfectant efficacy tests
Various regulatory authorities in Europe (e.g. European Standard or Norm, EN; British Standards, BS; Germany, DGHM; France, AFNOR) and North America (e.g. Food and Drug Administration, FDA; Environmental Protection Authority, EPA; Association of Official Analytical Chemists, AOAC) have been associated with attempts to produce some form of harmonization of dis-infectant tests. Perhaps the most readily accessible and recent guide to the methodology of possible bactericidal, tuberculocidal, fungicidal and viricidal disinfectant efficacy tests, is that of Kampf and colleagues (2002). This publication summarizes and provides references to various EN procedures (e.g. prEN 12054).
3.2.1 Suspension tests
While varying to some degree in their methodology, most of the proposed procedures tend to employ a standard suspension of the microorganism in hard water containing albumin (dirty conditions) and appropriate dilutions of the disinfectant—so-called suspension tests. Tests are carried out at a set temperature (usually around room temperature or 20°C), and at a selected time interval samples are removed and viable counts are performed following neutralization of any disinfectant remaining in the sample. Neutralization or inactivation of residual dis-infectant can be carried out by dilution, or by addition of specific agents (see Table 18.3). Using viable counts, it is possible to calculate the concentration of disinfectant required to kill 99.999% (5-log kill) of the original sus-pension. Thus 10 survivors from an original population of 106 cells represents a 99.999% or 5-log kill. As bacteria may initially decline in numbers in diluents devoid of additional disinfectant, results from tests incorporating disinfectant-treated cells can be compared with results from simultaneous tests involving a non-disinfectant-containing system (untreated cells). The bactericidal effect BE can then be expressed as:
Table 18.3 Neutralizing agents for some antimicrobial agents
Antimicrobial agent | Neutralizing and/or inactivating agentb |
Alcohols | None (dilution) |
Alcohol-based hand gels | Tween 80, saponin, histidine and lecithin |
Amoxycillin | β-Lactamase from Bacillus cereusc |
Antibiotics (most) | None (dilution, membrane filtration,d resin adsorptione) |
Benzoic acid | Dilution or Tween 80f |
Benzyl penicillin | β-Lactamase from Bacillus cereus |
Bronopol | Cysteine hydrochloride |
Chlorhexidine | Lubrol W and egg lecithin or Tween 80 and lecithin (Letheen) |
Formaldehyde | Ammonium ions |
Glutaraldehyde | Glycine |
Halogens | Sodium thiosulphate |
Hexachlorophane | Tween 80 |
Mercurials | Thioglycollic acid (-SH compounds) |
Phenolics | Dilution or Tween 80 |
QACs | Lubrol W and lecithin or Tween 80 and lecithin (Letheen) |
Sulphonamides | p-Aminobenzoic acid |
a Other than dilution.
b D/E neutralizing media—adequate for QACs, phenols, iodine and chlorine compounds, mecurials, formaldehyde and glutaraldehyde (see Rutala, 1999).
c O ther appropriate enzymes can be considered, e.g. inactivating or modifying enzymes for chloramphenicol and aminoglycosides, respectively.
d F ilter microorganisms on to membrane, wash, transfer membrane to growth medium.
e R esins for the absorption of antibiotics from fluids are available.
f Tween 80 (polysorbate 80).
Source: adapted from Hugo & Russell (1998).
(6)
where NC and ND represent the final number of CFU/ml remaining in the control and disinfectant series, respectively.
Unfortunately, viable count procedures are based on the assumption that one colony develops from one viable cell or one CFU. Such techniques are, therefore, not ideal for disinfectants (e.g. QACs such as cetrimide) that promote clumping in bacterial suspensions, although the latter problem may be overcome by adding nonionic surface active agents to the diluting fluid.
3.2.2 In-use and simulated use tests
Apart from suspension tests, in-use testing of used medical devices, and simulated use tests involving instruments or surfaces deliberately contaminated with an organic load and the appropriate test microorganism have been incorporated into disinfectant testing protocols. An example is the in-use test first reported by Maurer in 1972. It is used to determine whether the disinfectant in jars, buckets or other containers in which potentially contaminated material (e.g. lavatory brushes, mops) has been placed contain living microorganisms, and in what numbers. A small volume of fluid is withdrawn from the in-use container, neutralized in a large volume of a suitable diluent, and viable counts are performed on the resulting suspension. Two plates are involved in viable count investigations, one of which is incubated for 3 days at 32°C (rather than 37°C, as bacteria damaged by disinfectants recover more rapidly at lowered temperatures), and the other for 7 days at room temperature. Growth of one or two colonies per plate can be ignored (a disinfectant is not usually a sterilant), but 10 or more colonies would suggest poor and unsatisfactory cidal action.
Simulated use tests involve deliberate contamination of instruments, inanimate surfaces, or even skin surfaces, with a microbial suspension. This may either be under clean conditions or may utilize a diluent containing organic material (e.g. albumin) to simulate dirty condi-tions. After being left to dry, the contaminated surface is exposed to the test disinfectant for an appropriate time interval. The microbes are then removed (e.g. by rubbing with a sterile swab), resuspended in suitable neutralizing medium, and assessed for viability as for suspension tests. New products are often compared with a known comparator compound (e.g. 1 minute application of 60% v/v 2-propanol for hand disinfection products—see EN1500) to show increased efficacy of the novel product.
3.2.3 Problematic bacteria
Mycobacteria are hydrophobic in nature and, as a result, exhibit an increased tendency to clump or aggregate in aqueous media. It may be difficult, therefore, to prepare homogeneous suspensions devoid of undue cell clumping (which may contribute to their resistance to chemical disinfection). As Mycobacterium tuberculosis is very slow growing, more rapidly growing species such as M. terrae, M. bovis or M. smegatis can be substituted in tests (as representative of M. tuberculosis). Recent global public health concerns regarding the increasing incidences of tuberculosis (including co-infections with HIV) in devel-oping, middletier and industrialized nations brings into sharp focus the necessity for representative evaluations of agents with potential tuberculocidal activity. This is particularly true given the high proportion of cases classified as multidrug resistant tuberculosis (MDR-TB). Apart from vegetative bacterial cells, bacterial or fungal spores can also be used as the inoculum in tests. In such cases, incubation of plates for the final viability determination should be continued for several days to allow for germination and growth.
Compared with suspended (planktonic) cells, bacteria on surfaces as biofilms are invariably phenotypically more tolerant to antimicrobial agents. With biofilms, suspension tests can be modified to involve biofilms produced on small pieces of an appropriate glass, metal or polymeric substrate, or on the bottom of microtitre tray wells. After being immersed in, or exposed to, the disinfectant solution for the appropriate time interval, the cells from the biofilm are removed, e.g. by sonication, and resuspended in a suitable neutralizing medium. Viable counts are then performed on the resulting planktonic cells. Reduction in biomass following antimicrobial challenge can be monitored using a standard crystal violet staining technique, however, viable counting permits evaluation of rate of kill. The Calgary Biofilm Device, discussed in section 9.1, permits the high-throughput screening of antimicrobial agents against biofilms grown on 96 polycarbonate pegs in a 96-well microtitre plate. Some important environmental bacteria survive in nature as intracellular parasites of other microbes, e.g. Legionella pneumophila within the protozoan Acanthamoeba polyphaga. Biocide activity is significantly reduced against intracelluar legionellae (see Figure 18.3). Disinfectant tests involving such bacteria should therefore be conducted both on planktonic bacteria and on suspensions involving amoebae-containing bacteria. With the latter, the final bacterial viable counts are performed after suitable lysis of the protozoan host. The legionella/protozoa situation may also be further complicated by the fact that the microbes often occur as biofilms.
3.3 Other microbe disinfectant test
Suspension-type efficacy tests can also be performed on other microbes, e.g. fungi, viruses, using similar techniques to that described above for bacteria, although significant differences obviously occur in parts of the tests.
3.3.1 Antifungal (fungicidal) test
In order for disinfectants to claim fungicidal activity, or for the discovery of novel fungicidal activities, a range of standard tests have been devised. Perhaps the main problem with fungi concerns the question of which morphological form of fungi to use as the inoculum. Unicellular yeasts can be treated as for bacteria, but whether to use spores (which may be more resistant than the vegetative mycelium) or pieces of hyphae with the filamentous moulds, has yet to be fully resolved. Spore suspensions (in saline containing the wetting agent Tween 80) obtained from 7-day-old cultures are presently recommended. The species to be used may be a known environmental strain and likely contaminant, such as Aspergillus niger, or a pathogen, such as Trichophyton mentagrophyes, other strains such as Penicillium variabile are also employed. Clearly the final selection of organism will vary depending on the perceived use for the disinfectant under test. In general, spore suspensions of at least 106 CFU/ml have been recommended. Viable counts are typically performed on a suitable media (e.g. malt extract agar, sabouraud dextrose agar) with incubation at 20°C for 48 hours or longer. EN 1275:1997 regulations for fungicidal activity require a minimum reduction in viability by a factor of 104 within 60 minutes; test fungi were Candida albicans and A. niger. Further procedures may be obtained by reference to EN 1650:1998 (quantitative suspension test for evaluation of fungicidal activity of chemical disinfectants and antiseptics used in food, industrial, domestic and institutional areas) and AOAC Fungicidal activity of disinfectants (955.17).
3.3.2 Antiviral (viricidal) test
The evaluation of disinfectants for viricidal activity is a complicated process requiring specialized training and facilities; viruses are obligate intracellular parasites and are therefore incapable of independent growth and replication in artificial culture media. They require some other system employing living host cells. Suggested test viruses include rotavirus, adenovirus, poliovirus, herpes simplex viruses, HIV, pox viruses and papovavirus, although extension of this list to include additional blood-borne viruses such as hepatitis B and C, and significant animal pathogens (e.g. foot and mouth disease virus) could be argued, given the potential impact on public health or the economy of a nation.
Briefly, the virus is grown in an appropriate cell line that is then mixed with water containing an organic load and the disinfectant under test. After the appropriate time, residual viral infectivity is determined using a tissue culture/plaque assay or other system (e.g. animal host, molecular assay for some specific viral component). Such procedures are costly and time-consuming, and must be appropriately controlled to exclude factors such as disinfectant killing of the cell system or test animal. A reduction of infectivity by a factor of 104 has been regarded as evidence of acceptable viricidal activity (prEN 14476). For viruses that cannot be grown in the laboratory (e.g. hepatitis B), naturally infected cells/tissues must be used. Further test procedures are detailed in British Standard BS EN 13610 (quantitative suspension test for the evaluation of viricidal activity against bacteriophages of chemical disinfectants used in food and industrial areas). The use of bacteriophage as model viruses in this procedure most likely reflects their ease of growth and survivor enumeration via standard plaque assay on host bacterial lawns grown on solid media.
3.3.3 Prion disinfection test
Prions are a unique class of acellular, proteinaceous infectious agent, devoid of an agent-specific nucleic acid (DNA or RNA). Infection is associated with the abnormal isoform of a host cellular protein called prion protein (PrPc). Prions exhibit unusually high resistance to conventional chemical and physical decontamination methods, presenting a unique challenge in infection control. Although numerous published studies on prion inactivation by disinfectants are available in the literature, inconsistencies in methodology make direct comparison difficult. For example, strain differences of prion (with respect to sensitivity to thermal and chemical inactiva-tion), prion concentration in tissue homogenate, exposure conditions and determination of log reductions from incubation period assays instead of end-point titra-tions. Furthermore, since most studies of prion inactivation have been conducted with tissue homogenates, the protective effect of the tissue components may offer some protective role and contribute to resistance to disinfection approaches. Despite this, a consistent picture of effective and ineffective agents has emerged and is summarized in Table 18.4. Although most disinfectants are inadequate for the elimination of prion infectivity, agents such as sodium hydroxide, a phenolic formulation, guanidine thiocyanate and chlorine have all been shown to be effective.
Table 18.4 Efficacy of Chemical Agents in Prion Inactivation
Processes may be listed in both columns (ineffective/effective), due to different testing parameters or testing methods. All experiments conducted without prior cleaning. Adapted from Rutala & Weber, 2010
Ineffective (≤3 log10 reduction within 1 hour) | Effective (>3 log10 reduction within 1 hour of temperatures 20°C—55°C) |
Acetone | Alkaline detergent (specific formulations) |
Alcohol, 50–100% | Enzymatic detergent (specific formn) |
Ammonia, 1.0 M | Chlorine >1,000 ppm |
Alkaline detergent (specific formulations) | Copper, 0.5 mmol/L and H202, 100 mmol/L |
Chlorine dioxide, 50 ppm | Guanidine thiocyanate, >3 M |
Formaldehyde, 3.7% | Peracetic acid, 0.2% |
Glutaraldehyde, 5% | Phenolic disinfectant (specific formn), >0.9% |
Hydrochloric acid, 1.0 N | QAC (specific formulation) |
Hydrogen peroxide, 0.2%–60% | Hydrogen peroxide, 59% |
Iodine, 2% | SDS, 2% and acetic acid, 1% |
Ortho-phthaladehyde, 0.55% | Sodium hydroxide, ≥1 N |
Peracetic acid, 0.2%–19% | Sodium metaperiodate, 0.01 M |
Phenol/phenolics (concn variable) | |
Potassium permanganate, 0.1%–0.8% | |
QAC (specific formulation) | |
Sodium dodecyl sulfate (SDS) 1%–5% Sodium deoxycholate, 5% | |
Enzymatic detergent (specific formulations) | |
Triton X-100, 1% | |
Urea, 4–8 M |
4 Evaluation of solid disinfectants
Solid disinfectants usually consist of a disinfectant substance diluted by an inert powder. Phenolic substances adsorbed onto kieselguhr (diatomite) form the basis of many disinfectant powders; another widely used solid disinfectant is sodium dichloroisocyanurate. Other disinfectant or antiseptic powders used in medicine include acriflavine and compounds with antifungal activity such as zinc undecenoate or salicylic acid mixed with talc. These disinfectants may be evaluated by applying them to suitable test organisms growing on a solid agar medium. Discs may be cut from the agar and subcultured for enumeration of survivors. Inhibitory activity is evaluated by dusting the powders onto the surface of seeded agar plates, using the inert diluents as a control. The extent of growth is then observed following incubation.
5 Evaluation of air disinfectants
The decontamination and disinfection of air is an important consideration for both infection and contamination control. A large number of important infectious diseases are spread via microbial contamination of the air. This cross-infection can occur in a variety of situations (hos-pitals and care facilities, airplanes, public and institutional buildings), while stringent control of air quality with respect to airborne contaminants and particulates is critical for contamination control in many aseptic procedures. With the increasing public concern regarding the perceived heightened threat of bioterrorism, effective air disinfection procedures have been reviewed as a potential counter-measure. The microorganisms themselves may be contained in aerosols, or may occur as airborne particles liberated from some environmental source, e.g. agitation of sporeladen bed linen, decaying vegetation, etc. Disinfection of air can be carried out by increased ventila-tion, filtration of air through high-efficiency particulate air (HEPA) filters, chemical aerosol/vapour/fumigation or by ultraviolet germicidal irradiation (UVGI). Although UVGI disinfectant approaches have demonstrated efficacy against a range of airborne pathogens and contaminating organisms, it is often more practical to use some form of chemical vapour or aerosol to kill them. The use of formaldehyde vapour is the most commonly employed agent for fumigation procedures (not strictly air disinfection), although vaporized hydrogen peroxide may be used as an alternative agent. Due to the potential for formation of carcinogenic bis(chloromethyl) ether when used with hydrochloric acid and chlorine containing disinfectants, formaldehyde should not be used with hypochlorites.
The work of Robert Koch in the late 1880s demonstrated that the numbers of viable bacteria present in air can be assessed by simply exposing plates of solid nutrient media to the air. Indeed, this same process is still exploited in environmental monitoring in the form of settle plates. Any bacteria that fall on to the plates after a suitable exposure time can then be detected following an appropriate period of incubation. These gravitational methods are obviously applicable to many microor-ganisms, but are unsuitable for viruses. However, more meaningful data can be obtained if force rather than gravity is used to collect airborne particles. A stream of air can be directed on to the surface of a nutrient agar plate (impaction; slit sampler) or bubbled through an appropriate buffer or culture medium (liquid impin-gement). Various commercial impactor samplers are available. Filtration sampling, where the air is passed through a porous membrane, which is then cultured, can also be used. For experimental evaluation of potential air disinfectants, bacterial or fungal airborne ‘suspensions’ can be created in a closed chamber, and then exposed to the disinfectant, which may be in the form of radiation, chemical vapour or aerosol. The airborne microbial population is then sampled at regular intervals using an appropriate forced-air apparatus such as the slit sampler. With viruses, the air can be bubbled through a suitable liquid medium, which is then subjected to some appropriate virological assay system. In all cases, problems arise in producing a suitable airborne microbial ‘suspension’ and in neutralizing residual disinfectant, which may remain in the air.
Preservatives are widely employed in the cosmetic and pharmaceutical industries as well as in a variety of other manufacturing industries. The addition of preservatives to pharmaceutical formulations to prevent microbial growth and subsequent spoilage, to retard product deterioration and to restrain growth of contaminating micro-organisms is commonplace for non-sterile pharmaceutical formulations as well as low-volume aseptically prepared formulations intended for multiple use from one con-tainer. Indeed, adequate preservation (and validation of effectiveness) is a legal requirement for certain formula-tions. Effective preservation prevents microbial and, as a consequence, related chemical, physical and aesthetic spoilage that could otherwise render the formulation unacceptable for patient use, therapeutically ineffective or harmful to the patient (due to presence of toxic metab-olites, microbial toxins). The factors which influence the activity of the cidal agent employed as a preservative are largely those which affect disinfectant activity (described in section 2.4), however, when considering the activity of the cidal agent the interactions with formulation components (adsorption to suspended particles, oil-water partitioning, etc.) should be considered as additional factors which can potentially attenuate the preservative activity.
While the inhibitory or cidal activity of the chemical to be used as the preservative can be evaluated using an appropriate in vitro test system (see sections 3.2.1 and 8.1.2), its continued activity when combined with the other ingredients in the final manufactured product must be established. Problems clearly exist with some products, where partitioning into various phases may result in the absence of preservative in one of the phases, e.g. oil-in-water emulsions where the preservative may partition only into the oily phase, allowing any contaminant microorganisms to flourish in the aqueous phase. In addition, one or more of the components may inactivate the preservative. Consequently, suitably designed simulated use challenge tests involving the final product are, therefore, required in addition to direct potency testing of the pure preservative. In the challenge test, the final preserved product is deliberately inoculated with a suitable environmental microorganism which may be fungal (e.g. C. albicans or A. niger)or bacterial (e.g. Staph. aureus, E. coli, Ps. aeruginosa). For oral preparations with a high sucrose content, the osmophilic yeast Zygosaccharomyces rouxii is a recommended challenge organism. The subsequent survival (inhibition), death or growth of the inoculum is then assessed using viable count techniques. Different performance criteria are laid down for injectable and ophthalmic preparations, topical preparations and oral liquid preparations in the British Pharmacopoeia (Appendix XVI C) and the European Pharmacopoeia, which should be consulted for full details of the experimental procedures to be used. In some instances, the range and/or spectrum of preservation can be extended by using more than one preservative at a time. Thus a combination of parabens (p-hydroxybenzoic acid) with varying water solubilities may protect both the aqueous and oil phases of an emulsion, while a combination of Germall 115 and parabens results in a preservative system with both antibacterial (Germall 115) and antifungal (parabens) activity.
In most of the tests mentioned above, results are not available until visible microbial growth occurs, at least in the controls. This usually takes 24 hours or more. The potential benefits of rapid antimicrobial susceptibility screening procedures are obvious, particularly in aggressive infections or rapidly progressing nosocomial infections of immunocompromised patients where appropriate antimicrobial selection is critical. To date only a few ‘rapid’ methods for detecting microbial viability or growth are presently employed in assessing the efficacy of antimicrobials. These include epifluorescent and bioluminescence techniques. The former relies on the fact that when exposed to the vital stain acridine orange and viewed under UV light, viable cells fluoresce green or greenish yellow, while dead cells appear orange. Live/dead staining of sessile bacterial populations has the potential to yield important data with respect to antimicrobial sus-ceptibility, but requires skilled personnel and specialized microscopy equipment.
With tests involving liquid systems the early growth of viable cells can be assessed by some lightscattering proc-esses, blood culture techniques have classically used the production of CO2 as an indicator of bacterial metabolism and growth. In addition, the availability of molecular techniques, such as quantitative PCR, may be useful in demonstrating the presence or growth of microorganisms that are slow or difficult to culture under usual laboratory conditions, e.g. viruses. This may obviate the need to neutralize residual disinfectant with some assays.
Recently, rapid colorimetric assays for antimicrobial susceptibility have been developed including the commercially available Vitek and Vitek2 systems (Biomerieux) and colourimetric tests based on the extracellular reduction of tetrazolium salts 2-(2-methoxy-4-nitrophenyl)-3-(4-nitrophenyl)-5-(2, 4-disulfophenyl)-2 H-tetrazolium monosodium (WST-8) and 2,3-bis[2-methyloxy-4-nitro-5-sulfophenyl]-2 H-tetrazolium-5-carboxanilide (XTT). These latter studies have demonstrated the potential for the tetrazolium salts WST-8 and XTT to be used in the rapid, accurate and facile screening of antimicrobial susceptibility and MIC determination in a range of bacteria, including staphylococci, extended β-lactamase producing clinical isolates (E. coli, Ent. faecalis) and Ps. aeruginosa (see Tunney et al., 2004). Using this method, MIC values in agreement with those obtained using standard methods were obtained after 5 hours.
8 Evaluation of potential chemotherapeutic antimicrobials
Unlike tests for the evaluation of disinfectants, where determination of cidal activity is of paramount impor-tance, tests involving potential chemotherapeutic agents (antibiotics) invariably have determination of MIC as their main focus. Tests for the bacteriostatic activity of antimicrobial agents are valuable tools in predicting antimicrobial sensitivity/tolerance in individual patient samples and for detection and monitoring of resistant bacteria. However, correlation between MIC and therapeutic outcome are frequently difficult to predict, especially in chronic biofilm-mediated infections. The determination of MIC values must be conducted under standardized conditions, since deviation from standard test conditions can result in considerable variation in data.
8.1 Tests for bacteriostatic activity
The historical gradient plates, ditch-plate and cup-plate techniques (see Hugo & Russell, 1998) have been replaced by more quantitative techniques such as disc diffusion (Figure 18.4), broth and agar dilution, and E-tests (Figure 18.5). All employ chemically defined media (e.g. Mueller-Hinton or Iso-Sensitest) at a pH of 7.2–7.4, and in the case of solid media, agar plates of defined thickness. Regularly updated guidelines have been provided by the National Committee for Clinical Laboratory Standards (NCCLS) and are widely used in many coun-tries, although the British Society for Antimicrobial Chemotherapy has produced its own guidelines and testing procedures (Andrews, 2009).
Figure 18.4 Disc test with inhibition zones around two (1, 2) of five discs. The zone around disc 1 is clear and easy to measure, whereas that around disc 2 is indistinct. Although none of the antimicrobials in discs 3, 4 or 5 appear to inhibit the bacterium, synergy (as evidenced by inhibition of growth between the discs) is evident with the antimicrobials in discs 3 and 5. Slight antagonism of the drug in disc 1 by that in disc 3 is evident.
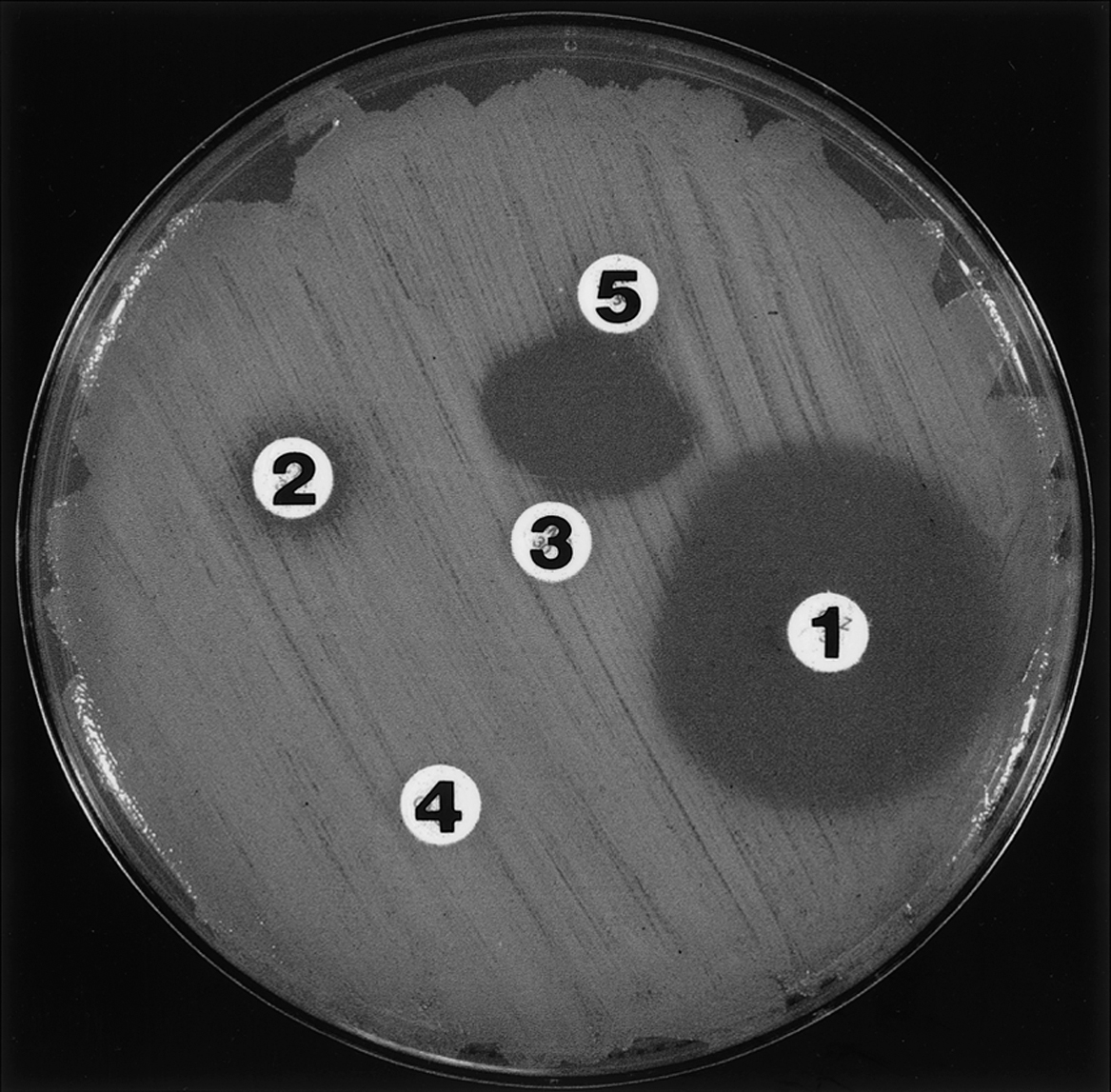
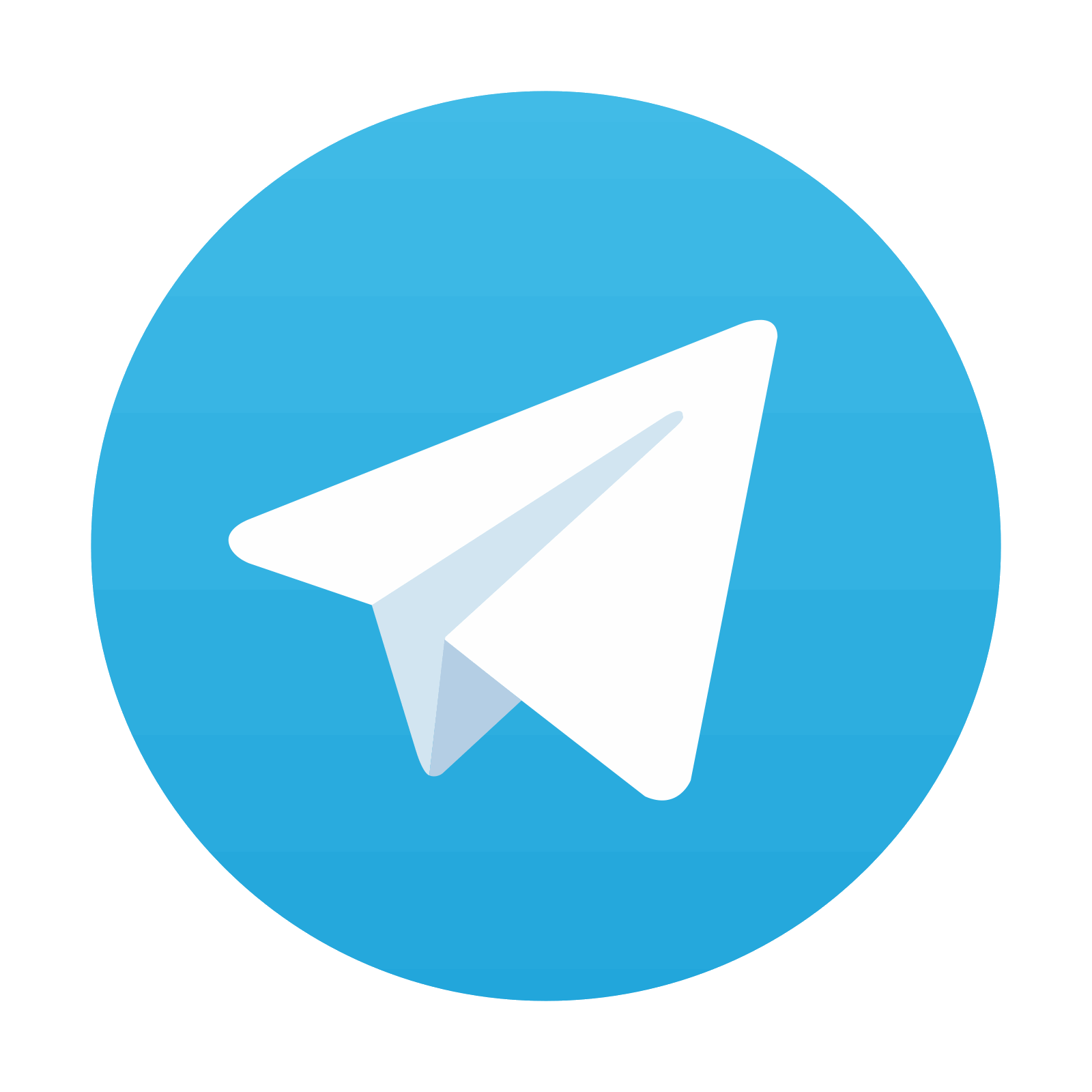