Histamine is a biogenic amine found in many tissues, including mast cells, basophils, lymphocytes, neurons, and gastric enterochromaffin-like cells. It is an autacoid—that is, a molecule secreted locally to increase or decrease the activity of nearby cells. Histamine is a major mediator of allergic and inflammatory processes: it also has significant roles in the regulation of gastric acid secretion, neurotransmission, and immune modulation. Knowledge of the diverse actions of histamine has led to the development of a number of widely used pharmacologic agents that regulate the effects of histamine in pathologic states. This chapter focuses on the pharmacologic actions of H1-antihistamines; H2-antihistamines are discussed in Chapter 47, Integrative Inflammation Pharmacology: Peptic Ulcer Disease.
Ellen, a generally healthy 76-year-old grandmother, suffers from allergic rhinitis. Every spring, she develops a runny nose, itchy eyes, and sneezing. To relieve her symptoms, she takes an over-the-counter antihistamine, diphenhydramine. She is annoyed by the unpleasant effects that accompany her allergy medication. Every time she takes her antihistamine, Ellen feels drowsy and her mouth feels dry. She makes an appointment with her doctor, who subsequently advises Ellen to take loratadine. Upon taking her new allergy medication, Ellen’s symptoms are relieved and she experiences no drowsiness or other adverse effects.
Questions
1. Why does Ellen develop seasonal allergic rhinitis?
2. What is the mechanism of action of diphenhydramine and loratadine?
3. Why does diphenhydramine cause drowsiness and dry mouth, and why doesn’t loratadine cause drowsiness or dry mouth?
Histamine Synthesis, Storage, and Release
Histamine is synthesized from the amino acid L-histidine. The enzyme histidine decarboxylase catalyzes the decarboxylation of histidine to 2-(4-imidazolyl)ethylamine, commonly known as histamine (Fig. 44-1). The synthesis of histamine occurs in mast cells and basophils of the immune system, enterochromaffin-like (ECL) cells in the gastric mucosa, and certain neurons in the central nervous system (CNS) that use histamine as a neurotransmitter. Oxidative pathways in the liver rapidly degrade circulating histamine to inert metabolites. One major metabolite of histamine, imidazole acetic acid, can be measured in the urine to determine the amount of histamine that has been released systemically.
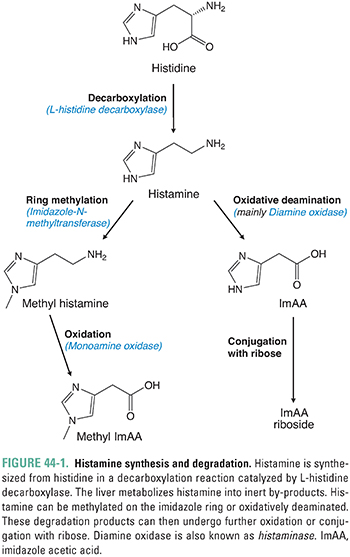
Histamine synthesis and storage can be divided into two “pools”: a slowly turning over pool and a rapidly turning over pool. The slowly turning over pool is located in mast cells and basophils. Histamine is stored in large granules in these inflammatory cells, and the release of histamine involves complete degranulation of the cells. Degranulation can be triggered by allergic processes, anaphylaxis, or cellular destruction from trauma, cold, or other insults. This pool is termed slowly turning over because several weeks are required to replenish the stores of histamine after degranulation has occurred. The rapidly turning over pool is located in gastric ECL cells and in histaminergic CNS neurons. These cells synthesize and release histamine as required for gastric acid secretion and neurotransmission, respectively. Unlike mast cells and basophils, ECL cells and histaminergic neurons do not store histamine. Instead, the production and release of histamine in these cells depend on physiologic stimuli. In the gut, for example, histidine decarboxylase is activated after the ingestion of food.
Histamine has a broad spectrum of actions involving many organs and organ systems. To understand the roles of histamine, it is useful to consider the physiologic effects of histamine in each tissue (Table 44-1). These effects include actions on bronchial smooth muscle, vascular smooth muscle, vascular endothelium, afferent nerve terminals, heart, gastrointestinal tract, and CNS.
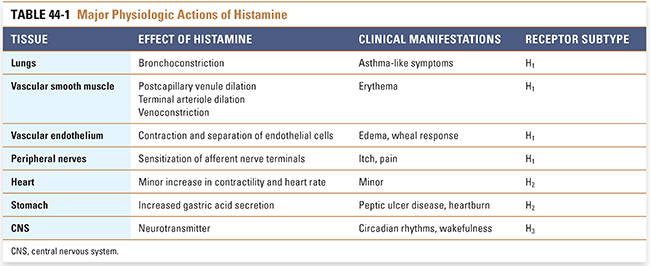
On smooth muscle, histamine causes some muscle fibers to contract and others to relax. In the human respiratory system, histamine causes bronchoconstriction (the effect varies in other species). However, the sensitivity of bronchial smooth muscle to histamine varies among individuals; those with asthma may be up to 1,000 times more sensitive to histamine-mediated bronchoconstriction than nonasthmatic individuals. Other smooth muscles—such as those in the bowel, bladder, iris, and uterus—also contract on exposure to histamine, but these effects are not thought to play a large role physiologically or clinically.
In vascular smooth muscle, histamine dilates postcapillary venules and terminal arterioles. Veins, however, constrict on exposure to histamine. The vasodilatory effect on the postcapillary venule bed is the most prominent effect of histamine on the vasculature. During infection or injury, histamine-induced venule dilation engorges the local microvasculature with blood, enhancing the access of immune cells that initiate repair processes to the damaged area. This engorgement explains the erythema observed in inflamed tissues.
Histamine also causes contraction of vascular endothelial cells. Histamine-induced endothelial cell contraction causes these cells to separate from one another, allowing release of plasma proteins and fluid from postcapillary venules and thereby causing edema. Thus, histamine is a key mediator of local responses at sites of injury.
Peripheral sensory nerve terminals also respond to histamine. The sensations of itch and pain result from a direct depolarizing action of histamine on afferent nerve terminals. This effect is responsible for the pain and itch experienced after an insect bite, for example.
The combined actions of histamine on vascular smooth muscle, vascular endothelial cells, and peripheral nerve terminals are responsible for the wheal-and-flare response noted after histamine release in the skin. Endothelial cell contraction causes the edematous wheal response, while the red, painful flare results from vasodilation and sensory nerve stimulation. Histamine also causes a similar process to occur in the nasal mucosa. Endothelial cell contraction, increased vascular permeability, glandular hypersecretion, and stimulation of irritant receptors contribute to mucosal edema and rhinorrhea, as well as the itching and sneezing typical of allergic rhinitis.
The cardiac effects of histamine consist of minor increases in the force and rate of cardiac contraction. Histamine enhances Ca2+ influx into cardiac myocytes, leading to increased inotropy. The increase in heart rate is caused by an increase in the rate of phase 4 depolarization in sinoatrial nodal cells.
The primary role of histamine in the gastric mucosa is to potentiate gastrin-induced acid secretion. Histamine is one of three molecules that stimulate acid secretion in the stomach, the others being gastrin and acetylcholine. Activation of histamine receptors in the stomach leads to an increase in intracellular Ca2+ in parietal cells and results in increased secretion of hydrochloric acid by the gastric mucosa.
Finally, histamine functions as a neurotransmitter in the CNS. Histaminergic neurons originate in the tuberomammillary nucleus of the hypothalamus and project diffusely throughout the brain and spinal cord. Although the functions of histamine in the CNS are not well understood, histamine is believed to be important in the maintenance of sleep–wake cycles (circadian rhythms), cognitive processes (attention, memory, and learning), and feeding behaviors (appetite suppression).
Histamine actions are mediated by the binding of histamine to one of four receptor subtypes: H1, H2, H3, and H4. All four subtypes are seven-transmembrane, G protein-coupled receptors, and all demonstrate constitutive activity independent of agonist binding. The receptor isoforms differ in their expression levels, second messenger pathways, and tissue distributions (Table 44-2).
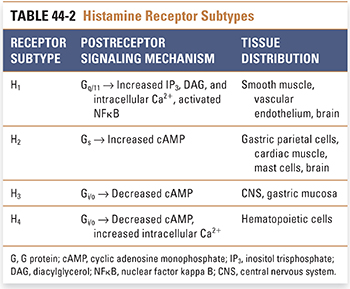
The H1 receptor activates G protein-mediated hydrolysis of phosphatidylinositol-4,5-bisphosphate (PIP2), leading to increased intracellular concentrations of inositol trisphosphate (IP3) and diacylglycerol (DAG). IP3 triggers the release of Ca2+ from intracellular stores, increasing cytosolic Ca2+ concentration and activating downstream pathways. DAG activates protein kinase C, leading to phosphorylation of numerous cytosolic target proteins. In some tissues, such as bronchial smooth muscle, the increase in cytosolic Ca2+ causes smooth muscle contraction by Ca2+/calmodulin-mediated activation of myosin light chain kinase, which phosphorylates myosin light chain. In other tissues, especially precapillary arteriolar sphincters and postcapillary venules, the increase in cytosolic Ca2+ causes smooth muscle relaxation by inducing the synthesis of nitric oxide (see Chapter 22, Pharmacology of Vascular Tone). H1 receptor stimulation also leads to the activation of NFκB, an important and ubiquitous transcription factor that promotes the expression of adhesion molecules and proinflammatory cytokines.
H1 receptors are expressed primarily on vascular endothelial cells and smooth muscle cells. These receptors mediate inflammatory and allergic reactions. Tissue-specific responses to H1 receptor stimulation include (1) edema, (2) erythema, (3) bronchoconstriction, and (4) sensitization of primary afferent nerve terminals. H1 receptors are also expressed on postsynaptic neurons in the tuberomammillary nucleus of the hypothalamus, cerebral cortex, and limbic system. These neurons appear to be involved in the control of circadian rhythms, wakefulness, and energy metabolism.
The major function of the H2 receptor is to mediate gastric acid secretion in the stomach. This receptor subtype is expressed on parietal cells in the gastric mucosa, where histamine acts synergistically with gastrin and acetylcholine to regulate acid secretion (see Chapter 47). H2 receptors are also expressed on cardiac muscle cells, on some immune cells, and on certain postsynaptic neurons in the CNS. H2 receptors on parietal cells activate a G protein-dependent cyclic AMP cascade, leading to enhanced proton pump-mediated delivery of protons into the gastric fluid.
Whereas H1 and H2 receptor subtypes have been well characterized, H3 and H4 receptor subtypes and their downstream actions are areas of active investigation. H3 receptors are predominantly located on presynaptic neurons in distinct regions of the CNS, including the cerebral cortex, basal ganglia, and tuberomammillary nucleus of the hypothalamus. H3 receptors appear to function as both autoreceptors and heteroreceptors, thereby limiting the synthesis and release of histamine as well as other neurotransmitters, including dopamine, acetylcholine, norepinephrine, GABA, and serotonin. This complex interaction between histamine and various neurotransmitter systems contributes to histamine’s widespread effects on CNS functions, including wakefulness, appetite, and memory. The downstream effects of H3 receptor activation are mediated via a decrease in cAMP.
H4 receptors are primarily localized to cells of hematopoietic origin, primarily mast cells, eosinophils, dendritic cells, and basophils. H4 receptors share 40% homology with H3 receptors and bind many H3 receptor agonists, although with lower affinity. Coupling of the H4 receptor to Gi/o leads to decreased cAMP and activation of phospholipase Cβ, and downstream events result in increased intracellular Ca2+. H4 receptors are of particular interest because they are thought to play an important role in inflammation; activation of H4 receptors mediates histamine-induced leukotriene B4 production, adhesion molecule up-regulation, and chemotaxis of mast cells, eosinophils, and dendritic cells. H4 receptors also appear to play a role in modulating itch and pain.
Histamine is an essential mediator of immune and inflammatory responses. Histamine plays a prominent role in the IgE-mediated type I hypersensitivity reaction, also known as the allergic reaction. In a localized allergic reaction, an allergen (antigen) first penetrates an epithelial surface (e.g., skin, nasal mucosa). The allergen can also be delivered systemically, as in the case of an allergic response to penicillin. With the aid of T-helper (TH) cells, the allergen stimulates B lymphocytes to produce IgE antibodies that are specific for that allergen. The IgE then binds to Fc receptors on mast cells and basophils, in a process known as sensitization. Once these immune cells are “sensitized” with IgE antibodies, they are able to detect and respond rapidly to a subsequent exposure to the allergen. Upon such an exposure, the allergen binds to and cross-links the IgE/Fc receptor complexes, triggering cell degranulation (Fig. 44-2).
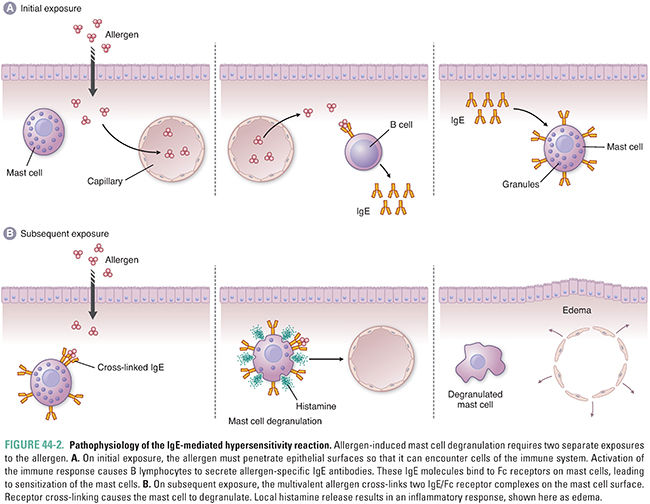
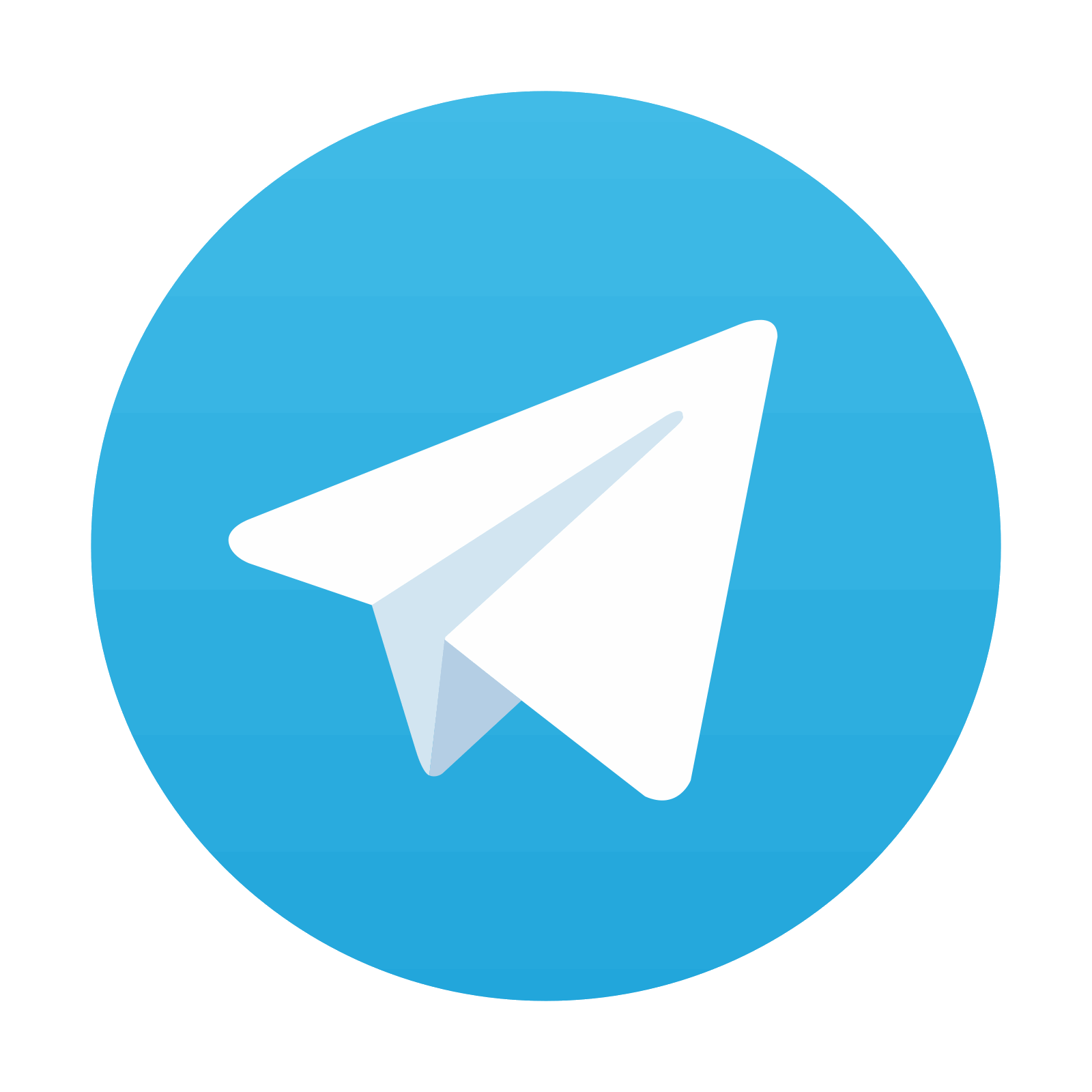
Stay updated, free articles. Join our Telegram channel
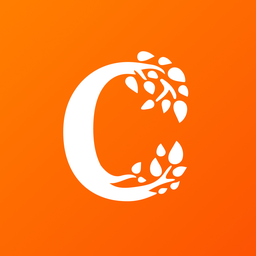
Full access? Get Clinical Tree
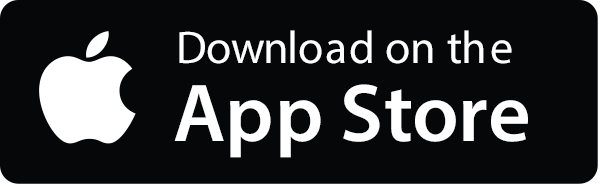
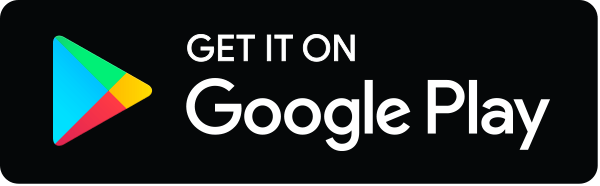