Fig. 1.1
Schematic illustration of hierarchical structure of bone ranging from the atomic to organ level
Bones are classified into cortical and spongy types, which are responsible mainly for load support and turnover, respectively. The relative ratio of spongy bone surface area to volume is greater than that of cortical bone since it contributes to essential mineral homeostasis through the bone marrow.
Figure 1.2 shows the anatomical median section of the human proximal femoral bone. The trabeculae in the maximum principal stress vector are preferentially aligned in abundant spongy bone at the end of cortical bone according to Wolff’s law [7, 8]. From the viewpoint of trabecular direction, the areas of spongy bone within bony trabeculae of the proximal femur are classified into the following groups based on the dominant principal stress direction: greater trochanter group; secondary compressive group; secondary tensile group; principal tensile group; principal compressive group; and Ward’s triangle, a radiolucent area between the principal compressive, secondary compressive, and principal tensile trabeculae near the neck of the femur that features low-density trabeculae [9].
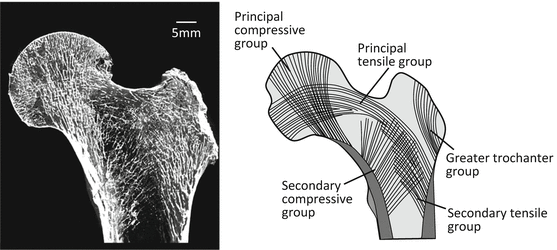
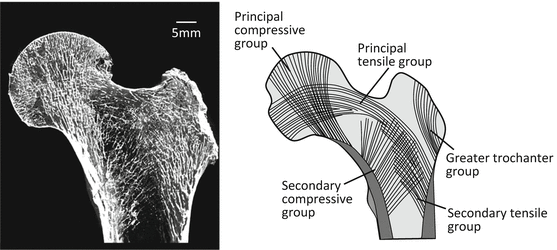
Fig. 1.2
Schematic illustration of typical trabecular pattern in the proximal portion of a human femur. The directions of the trabecular extension are well correlated with the in vivo stress pattern
Bone dynamics are regulated by bone cell function and the related abundant blood vessels and nerves. A cylindrical unit called the osteon forms multiple concentric circles in the Haversian canal through which a blood vessel traverses longitudinally in a long bone (Fig. 1.3) [10]. The internal system remains among the newly formed osteons in the osteal remodeling process.
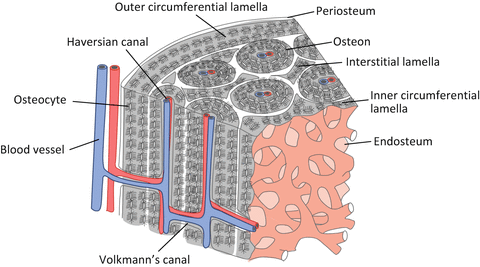
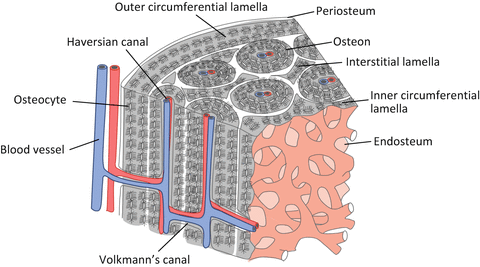
Fig. 1.3
Schematic illustration of the basic microstructure of a cortical bone
Furthermore, the diaphysis is surrounded by the outer circumferential lamellae just below the periosteum, while inner circumferential lamellae are located just below the endosteum. The nucleation site of calcification for each osteon is heterogeneous. The Haversian canal runs almost parallel to the bone longitudinal axis and connects with Volkmann’s canal and then produces the extensive blood vessel network within the cortical bone [11].
Bone is an internal organ with a very complicated structure that is difficult to mimic artificially. Therefore, bone replacement materials or an artificial joint including metal is used to support functional degradation of bone or bony joints. However, there is a significant difference in microstructure and related function between artificial materials and bone. Therefore, bone repair and artificial joint replacement must place great importance on mechanical safety and joint mobility but may result in only partial bone function recovery. In addition, a bone regenerative technique is also being developed that can reconstruct original bone tissue [12].
Diagnosis and treatment in orthopedic surgery are mainly performed according to bone mineral density (BMD) and bone mass. Therefore, new biomaterials and biodevices for bone development or bone joint replacement should consider the hierarchical and complicated organization of bone tissue containing anisotropic microstructure.
1.2.2 Bone Cells
The three dominant bone cell types, namely, osteoblasts, osteoclasts, and osteocytes, interact with other bone-related cells such as mesenchymal stem cells (Fig. 1.4) [13].
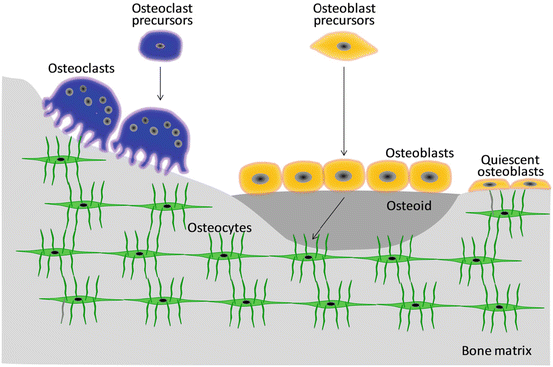
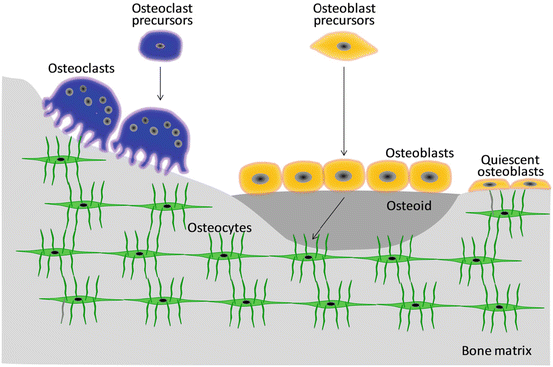
Fig. 1.4
Bone cells involved in bone modeling/remodeling
Osteoblasts are primarily arranged in a single layer on the surface of both cortical and spongy bones and exhibit an oval-like morphology that covers the bone surface like epithelial cells. Known as active as opposed to quiescent osteoblasts, they preferentially exist on the bone surface for active bone formation and possess cytoplasm, a localized cell nucleus, and a Golgi body near the cell nucleus. Active osteoblasts secrete non-collagen proteins such as osteocalcin and osteopontin and cytokines such as transforming growth factor-β (TGF-β) and bone morphogenetic protein (BMP). Furthermore, matrix vesicles are secreted that calcify the collagen fibril. Calcification of the bone tissue proceeds through the matrix vesicle-mediated process and mineralization along collagen fibers, both of which are preceded by active osteoblast [14].
As bone formation activity weakens, osteoblasts flatten and change into quiescent osteoblasts (bone-lining cells). Quiescent osteoblasts communicate with osteocytes through gap junctions and form well-developed networks. In such networks, osteoblasts become involved in the acceptance of various signal transduction pathways induced by the stimuli including mechanical stress, hormones, and cytokines and play a role in the regulation of mineral metabolism in collaboration with osteocytes. Runx2 (runt-related transcription factor 2) and Osterix are transcription factors that promote osteoblast differentiation through a BMP cascade [15].
Osteoblasts buried within the newly formed bone matrix subsequently change into osteocytes due to calcification of the surrounding pericellular matrix. Bone canaliculi connect the adjacent osteocyte lacunae. Osteocytes have anisotropic morphology and are surrounded by an anisotropically developed network. The bone canaliculi network is saturated by an interstitial fluid and is very important for the transport of solutes such as cytokines and metabolic products [16]. Osteocytes are the main receptor cells that detect mechanical stimuli and quickly transmit information to osteoblasts and osteoclasts through canaliculi. Sclerostin, a protein encoded by the SOST gene in osteocytes, suppresses bone formation by inhibiting Wnt signaling pathway [17]. Furthermore, fibroblast growth factor 23 (FGF-23) is produced in relation to sclerostin and suppresses phosphorous reabsorption by the kidney [18].
Osteoclasts, which have 5–10 nuclei and are 20–100 μm in diameter, control bone dissolution and absorption. The ruffled border, a deeply indented cell membrane, develops on the surface and dissolves bone mineral and organic bone constituents using acid and hydrolase, respectively, which are secreted by osteoclasts.
Osteoblasts, osteocytes, and osteoclasts work together to achieve bone homeostasis [19]. Bone tissue exhibits complicated functions derived from its hierarchical anisotropic microstructure. The intercellular communication between bone cells is extremely important for functional expression, as is parathyroid hormone and the nervous system, which are closely related to bone metabolic turnover [20]. Clarifying the mutual interaction based on the molecular biology of the cell–cell coupling is necessary to understand both bone formation and the promotion of bone repair when a bone defect is introduced.
1.2.3 Bone Fracture and Natural Healing
When a bone fracture occurs, small defects can be cured spontaneously. Figure 1.5 demonstrates the repair process of general bone fractures in terms of the macroscopic changes. The bone fracture healing process is classified into three phases: inflammatory, reparative, and remodeling [21].
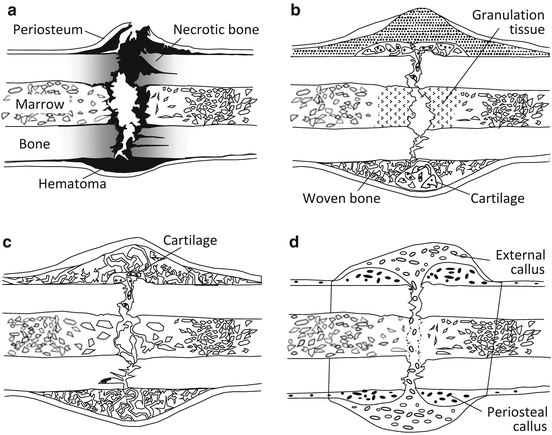
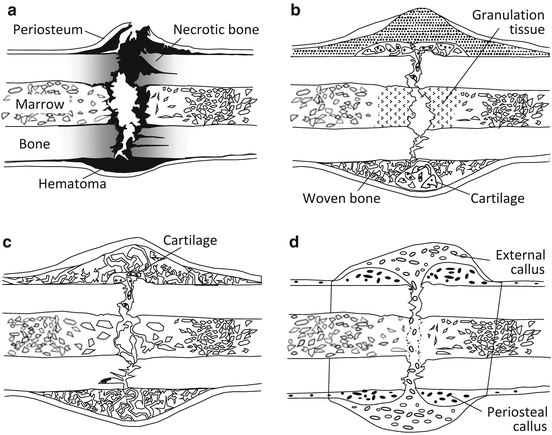
Fig. 1.5
Typical fracture healing process: (a) inflammatory phase, (b) reparative phase, (c) remodeling phase, and (d) bone union by external callus formation
In the inflammatory phase, in addition to the bone damage of the periosteum and bone marrow, the fractured space is occupied by a hematoma, and osteonecrosis occurs due to osteocyte necrosis and extinction near the broken end of the bone. An inflammatory reaction occurs in which the hematoma is removed, inflammatory granulation tissue forms, and osteoclasts remove necrotic bone (Fig. 1.5a).
The reparative phase consists of the soft callus phase and subsequent hard callus phase [22]. In the soft callus phase, capillary blood vessels invade and cells infiltrate the hematoma, the hematoma is removed, and granulation tissue is substituted. The hard callus phase is classified into endochondral and intramembranous ossification according to whether it occurs through cartilage [23]. Intramembranous ossification induces mineral deposition into a callus just under the periosteum without the appearance of chondrocytes when fixation is strong enough in the bone fracture region depending on mechanical stability [24]. In contrast, endochondral ossification proceeds via chondrocytes during calcification. In the remodeling phase, immature bone is substituted for lamellar bone due to remodeling, and the bone shape is gradually corrected to the original shape accompanied by marrow cavity formation (Fig. 1.5c). Bone union is finally achieved and the healing process is complete (Fig. 1.5d).
The bone reconstruction process proceeds when accompanied by the release of functional proteins such as BMP [25]. This process is characterized by simultaneous changes in macroshape and microstructure. Thus, changes of bone tissue from the macroscopic shape to the nanostructure level should be hierarchically understood during the process of bone fracture/repair or bone disease from the viewpoint of materials and structural parameters.
1.3 Bone Anisotropy
1.3.1 Anisotropy of Bone Mechanical Properties
Natural creatures under a gravitational environment have anisotropic and multiscale structures that exert efficient and anisotropic functions in the required direction. In other words, living beings can demonstrate the absolutely imperative ability to the fullest in a certain direction [26]. Bone is a typical anisotropic product.
In the human femoral shaft, bone strength strongly depends on the angle from the longitudinal bone axis in tension (Fig. 1.6) [27]. The anisotropy is clear in the stress–strain curves in Fig. 1.6, and the highest ultimate strength is seen parallel to the longitudinal bone axis but rapidly decreases as it approaches the vertical direction. A similar tendency is conspicuously seen in Young’s modulus and yield stress, and it is clear that a mechanical function adapts itself to the bone direction and is correlated to the applied in vivo stress. The anisotropy of a bone, represented by mechanical anisotropy in particular, is concerned with the anisotropic structure at the various size levels as mentioned in Sect. 1.2. Therefore, this section focuses upon microstructural anisotropy as it correlates with the mechanical, biological, and chemical functions of bone.
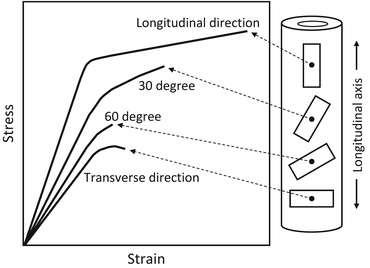
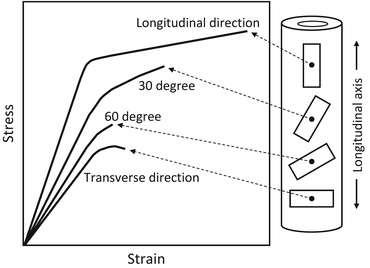
Fig. 1.6
Anisotropic biomechanical property of a long bone. Bone specimens taken in different orientations within the human cortical femur show different stress–strain behaviors (Redrawn from Frankel and Nordin [27])
1.3.2 Bone Anisotropic Structure in Intact Bones
1.3.2.1 Bone Microstructure Based on Apatite/Collagen Orientation
The development of biomaterials and medical devices depends on a clear understanding of the mechanical functions, related microstructure, and microenvironment of bone. Since the consensus statement by the US National Institutes of Health in 2000, bone quality has received attention as a factor determining bone strength as opposed to the traditional indicators of BMD and bone mass [28]. In 2000, proposed bone quality candidates included bone microstructure formation and restoration—typically, the trabecular network structure—and microcracks, collagen status, bone turnover, and cell viability. However, other essential controlling factors for bone quality focus on bone anisotropic microstructure [29, 30].
BAp crystallizes in an anisotropic hexagonal crystal system as an ionic crystal as shown in Fig. 1.7, and its base unit resembles a hexagonal pencil. The arrangement of ions is quite different along the a– and c-axes in the BAp crystallite, as the c-axis is parallel to the hexagonal pencil tip (Fig. 1.7) [5]. As a result, various bone functions are expected depending on the crystallographic direction such as the a– and c-axes [31]. Moreover, BAp crystallite is roughly distributed along the extending collagen fibril in bone, resulting in enhanced bone anisotropy [32]. Formation of the BAp/collagen complex with an anisotropic arrangement must be closely related to bone function. However, in terms of the present bone diagnosis, BMD and bone mass are generally analyzed by X-ray radiography and X-ray computed tomography (CT) method from the principle of X-ray absorption. In this case, the bone tissue anisotropy based on the BAp/collagen complex is undetectable.
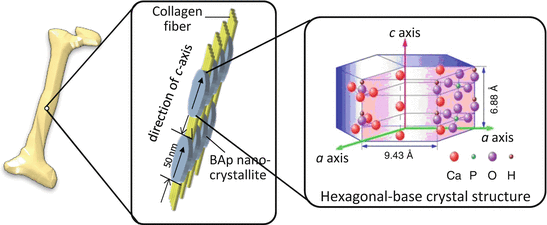
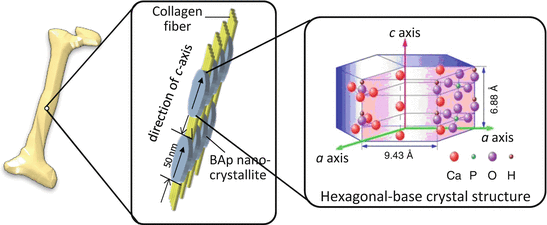
Fig. 1.7
Preferential orientation of biological apatite (BAp) nanocrystallite and collagen fiber in a long bone. The BAp that shows an anisotropic hexagonal crystal structure crystallizes epitaxially on the collagen fiber, where its c-axis is almost parallel to the collagen fiber direction
If there is no preferential three-dimensional arrangement of the BAp/collagen complex, the orientation of bone does not depend on its direction and does not influence the bone characteristics. However, because of the strong hierarchical anisotropy of bone, the preferred orientation degree and directional characteristics as a bone quality indicator should be considered in addition to BMD and bone mass as quantitative indices of BAp.
1.3.2.2 Analysis of Anisotropic Biological Apatite (BAp) Crystallite Using Microbeam X-Ray Diffraction (μXRD)
The preferential orientation of the BAp c-axis is roughly related to the direction of extended collagen and can be evaluated by various μXRD systems with collimators that are several dozen or hundreds of micrometers in diameter. Such systems are equipped with two-dimensional (2D) detectors (Fig. 1.8) [33]. One-dimensional (1D) or 2D detectors are generally used because the diffracted beam from the small volume of bone specimen is very weak, while the detected diffracted X-ray beam does not come from the symmetrical condition against the specimen surface. In addition, the preferential orientation of the collagen fibrils can be determined using micro-Raman spectroscopy by rotating the specimens with respect to polarization of the incident laser beam. Collagen molecules exhibit characteristic optical features that can aid in the identification of collagen fiber orientation by referring to the intensity between certain Raman bands, e.g., the amide I (~1,664 cm−1) and C–H bending (~1,451 cm−1) bands [34].
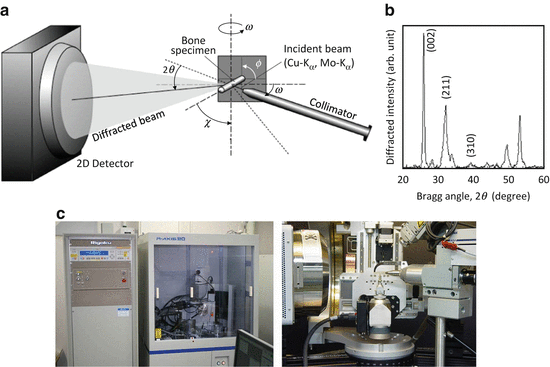
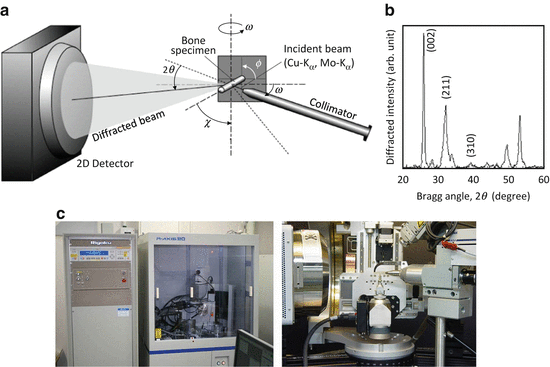
Fig. 1.8
Microbeam X-ray diffraction (μXRD) system for analyzing the biological apatite (BAp) orientation within bones: (a) schematic drawing of the transmission optical system, (b) X-ray diffraction peak profile taken from a long bone specimen along the long axis, and (c) pictures of the μXRD system developed by Rigaku (right) and Bruker AXS (left)
Figure 1.8 shows a schematic illustration of the transmission optics in the Bruker D8 with GADDS system. In this case, diffracted beams were simultaneously detected by a 2D position-sensitive proportional counter (2D PSPC) in which the intensity of the diffracted beam could be detected along the χ and 2θ axes. Distances between the specimen and collimator and between the specimen and detector are very important to the detectable resolution of diffracted beams within the detector. The focused incident beam with characteristic X-ray is used with wavelength of 0.15418 or 0.07107 nm for a Kα ray of copper or molybdenum, respectively. The balance between specimen width and penetration ability is very important, and the latter increases as wavelength decreases.
The specimens are fixed on an Eulerian goniometer with an X-Y-Z stage. The ϕ axis was closest to the specimen and provided complete specimen rotation around that axis. The χ-axis, the next closest to the specimen, was used to tilt the specimen. The specimens were moved to their desired positions by the X-Y-Z stage. The distribution of the X-ray absorption of the bone specimens was measured by an X-ray scintillation counter operating in the integrating mode [35].
Two diffraction peaks of hexagonal-based BAp, (002) and (310), are typically used to analyze BAp c-axis orientation [30]. The (002) and (310) diffraction peaks appear via a Cu-Kα incident beam around Bragg angles of 25.9° and 39.8°, respectively (Fig. 1.8b), when the cortical long bone is applied along the bone longitudinal axis. The peak positions of the (002) and (310) diffractions are changed depending on the wavelength of the incident beam into 11.9° and 18.1°, respectively, by the Mo-Kα beam. The degree of BAp c-axis orientation is defined to be an integrated intensity ratio of the (002) diffraction to the (310) diffraction.
1.3.2.3 Cortical Bone
The degree of BAp orientation in the bone structure corresponding to the intensity ratio of (002) to (310) changes substantially depending on the bone position and condition, even in the case of normal bones [30]. Figure 1.9 shows the degree of BAp c-axis orientation depending on the bone axis and position in various mature cortical bones analyzed using μXRD [30]. In the case of non-orientation, the relative XRD intensity ratio of (002)/(310) is approximately 2, indicating that in cases with higher diffraction intensity, it shows a preferential orientation of the BAp c-axis. In mature cortical bone structures, BMD does not change significantly, but BAp shows 1D preferential alignment with the c-axis arranged with the priority along the bone longitudinal, mesiodistal, and craniocaudal directions in the long bone of the rabbit ulna, monkey mandible, and monkey lumbar vertebra, respectively, within the cortical bone portions. On the other hand, a flat bone of the rabbit cranial bone shows a 2D orientation along the bone surface. The characteristic orientation distribution is closely related to the in vivo stress condition and bone growth process during normal bone turnover. In particular, the direction with the highest degree of BAp c-axis orientation is associated with the maximum principal stress direction in bones [30].
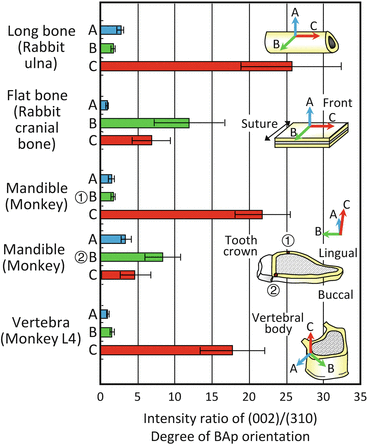
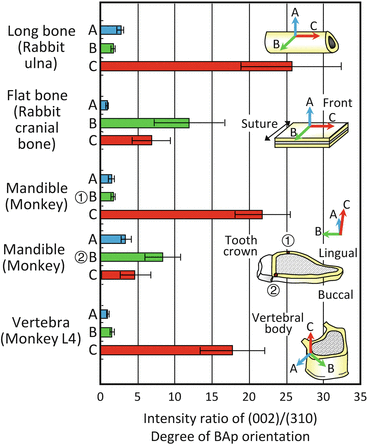
Fig. 1.9
The biological apatite (BAp) orientation distribution assessed as the relative intensity ratio of (002)/(310) in the long bone of a rabbit ulna, flat bone of a rabbit cranial bone, monkey mandible, and monkey vertebra. The BAp orientation largely varies depending on the bone type and direction despite similar bone mineral density (Reprinted from Ref. [30], Copyright 2002, with permission from Elsevier)
The monkey mandible essentially shows the BAp orientation along the mesiodistal direction (the C orientation), but just below the crown of the tooth, the preferential orientation of the BAp c-axis changes to the mastication direction (the B orientation) due to the biting direction [30]. This tendency is more remarkable on the buccal side than on the lingual side, which is easily exposed to mastication stress, and it controls the BAp orientation by handling the complex local changes in the in vivo stress distribution due to mastication [30, 36].
1.3.2.4 Spongy Bone
Preferential alignment of BAp c-axes is observed even inside each trabecula of spongy bone despite high turnover rate [37, 38].
Figure 1.10 shows preferential distribution of BAp orientation for a trabecula in a human lumbar vertebra analyzed detected using 2D PSPC as shown in Fig. 1.8a [37]. Bright circles or arcs correspond to the diffraction peak, and the (002) diffraction peak that shows the c-axis (c-plane) of BAp crystallite shows bright arcing along the extended direction of the trabecula. At the same time, the (310) diffraction intensity increases perpendicularly to the trabecular direction. This means that the trabeculae have a 1D orientation relationship of the preferred BAp c-axis along the extended direction similar to the cortical portion of long bone with a 1D orientation. This finding suggests that the trabecular bone is strengthened by the combination of the BAp preferential orientation and extending trabecular morphology along the maximum principal stress direction in vivo as mentioned in Fig. 1.2.
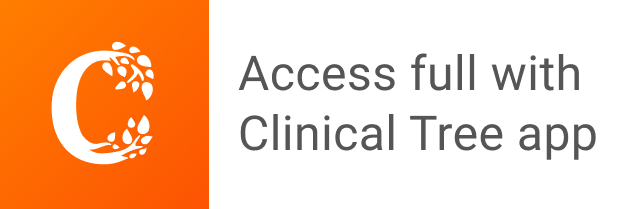