1.Introduction
The small1est free-living microorganisms are the prokaryotes, comprising bacteria and archaea (see Chapter 2). Prokaryote is a term used to define cells that lack a true nuclear membrane; they contrast with eukaryotic cells (e.g. plants, animals and fungi) that possess a nuclear membrane and internal compartmentalization. Indeed, a major feature of eukaryotic cells, absent from prokaryotic cells, is the presence in the cytoplasm of membrane-enclosed organelles. These and other criteria differentiating eukaryotes and prokaryotes are shown in Table 2.1.
Bacteria and archaea share many traits and it was not until the early 1980s that differences first became evident from analyses of gene sequences. One major difference is the composition of cell walls. A more striking contrast is in the structure of the lipids that make up their cytoplasmic membranes. Differences also exist in their respective patterns of metabolism: most archaea are anaerobes, and are often found inhabiting extreme environments. It is possible that their unusual membrane structure gives archaeal cells greater stability under extreme conditions. Of notable interest is the observation that no disease-causing archaea have yet been identified; the vast majority of prokaryotes of medical and pharmaceutical significance are bacteria.
Bacteria represent a large and diverse group of microorganisms that can exist as single cells or as cell clusters. Moreover, they are generally able to carry out their life processes of growth, energy generation and reproduction independently of other cells. In these respects they are very different from the cells of animals and plants, which are unable to live alone in nature and can exist only as part of a multicellular organism. They are capable of growing in a range of different environments and can not only cause contamination and spoilage of many pharmaceutical products but also a range of different diseases. For this reason, only bacteria are referred to throughout the remainder of this chapter.
1.1 Bacterial diversity and ubiquity
Bacterial diversity can be seen in terms of variation in cell size and shape (morphology), adaptation to environmental extremes, survival strategies and metabolic capabilities. Such diversity allows bacteria to grow in a multiplicity of environments ranging from hot sulphur springs (65°C) to deep freezers (−20°C), from high (pH 1) to low (pH 13) acidity and high (0.7 m) to low osmolarity (water). In addition, they can grow in both nutritionally rich (compost) and nutritionally poor (distilled water) situations. Hence, although each organism is uniquely suited to its own particular environmental niche and rarely grows out of it, the presence of bacteria may be considered ubiquitous. Indeed, there is no natural environment that is free from bacteria. This ubiquity is often demonstrated by terms used to describe organisms that grow and/or survive in particular environments. An example of such descriptive terminology is shown in Table 3.1.
Table 3.1 Descriptive terms used to describe bacteria
Descriptive term | Adaptive feature |
Psychrophile | Growth range −40°C to +20°C |
Mesophile | Growth range +20°C to +40°C |
Thermophile | Growth range +40°C to +85°C |
Thermoduric | Endure high temperatures |
Halophile | Salt-tolerant |
Acidophile | Acid-tolerant |
Aerobe | Air (oxygen) requiring |
Obligate anaerobe | Air (oxygen) poisoned |
Autotroph | Utilizes inorganic material |
Heterotroph | Requires organic material |
2.1 Cell size and shape
Bacteria are the smallest free-living organisms, their size being measured in micrometres (microns). Because of this small size a microscope affording a considerable degree of magnification (×400–1000) is necessary to observe them. Bacteria vary in size from a cell as small as 0.1–0.2μm in diameter to those that are >5μm in diameter. Bacteria this large, such as Thiomargarita namibiensis, are extremely rare: the majority of bacteria are 1–5 μm long and 1–2 μm in diameter. By comparison, eukaryotic cells may be 2 μ m to >200μ m in diameter. The small size of bacteria has a number of implications with regard to their biological properties, most notably increased and more efficient transport rates. This advantage allows bacteria far more rapid growth rates than eukaryotic cells.
While the classification of bacteria is immensely complex, nowadays relying very much on 16S ribosomal DNA sequencing data, a more simplistic approach is to divide them into major groups on purely morphological grounds. The majority of bacteria are unicellular and possess simple shapes, e.g. round (cocci), cylindrical (rod, also called bacillus, spelt with a lower case initial letter to distinguish from Bacillus, the genus) or ovoid. Some rods are curved (vibrios), while longer rigid curved organisms with multiple spirals are known as spirochaetes. Rarer morphological forms include the actinomycetes which are rigid bacteria resembling fungi that may grow as lengthy branched filaments; the mycoplasmas which lack a conventional peptidoglycan (murein) cell wall and are highly pleomorphic organisms of indefinite shape; and some miscellaneous bacteria comprising stalked, sheathed, budded and slime-producing forms often associated with aquatic and soil environments.
Often bacteria remain together in specific arrangements after cell division. These arrangements are usually characteristic of different organisms and can be used as part of a preliminary identification. Examples of such cellular arrangements include chains of rods or cocci, paired cells (diplococci), tetrads and clusters.
2.2 Cellular components
Compared with eukaryotic cells, bacteria possess a fairly simple base cell structure, comprising cell wall, cytoplasmic membrane, nucleoid, ribosomes and occasionally inclusion granules (Figure 3.1). Nevertheless it is important for several reasons to have a good knowledge of these structures and their functions. First, the study of bacteria provides an excellent route for probing the nature of biological processes, many of which are shared by multicellular organisms. Secondly, at an applied level, normal bacterial processes can be customized to benefit society on a mass scale. Here, an obvious example is the large-scale industrial production (fermentation) of antibiotics. Thirdly, from a pharmaceutical and healthcare perspective, it is important to be able to know how to kill bacterial contaminants and disease-causing organisms. To treat infections antimicrobial agents are used to inhibit the growth of bacteria, a process known as antimicrobial chemotherapy. The essence of antimicrobial chemotherapy is selective toxicity (Chapters 11, 12, 14 and 15), which is achieved by exploiting differences between the structure and metabolism of bacteria and host cells. Selective toxicity is, therefore, most efficient when a similar target does not exist in the host. Examples of such targets will be noted in the following sections.
Figure 3.1 Diagram of a bacterial cell. Features represented above the dotted line are only found in some bacteria, whereas those below the line are common to all bacteria.
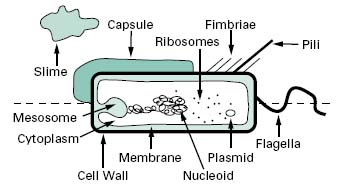
2.2.1 Cell wall
The bacterial cell wall is an extremely important structure, being essential for the maintenance of the shape and integrity of the bacterial cell. It is also chemically unlike any structure present in eukaryotic cells and is therefore an obvious target for antibiotics that can attack and kill bacteria without harm to the host (Chapter 12).
The primary function of the cell wall is to provide a strong, rigid structural component that can withstand the osmotic pressures caused by high chemical concentrations of inorganic ions in the cell. Most bacterial cell walls have in common a unique structural component called peptidoglycan (also called murein or glycopeptide); exceptions include the mycoplasmas, extreme halophiles and the archaea. Peptidoglycan is a large macromolecule containing glycan (polysaccharide) chains that are cross-linked by short peptide bridges. The glycan chain acts as a backbone to peptidoglycan, and is composed of alter-nating residues of N-acetyl muramic acid (NAM) and N-acetyl glucosamine (NAG). To each molecule of NAM is attached a tetrapeptide consisting of the amino acids L-alanine, D-alanine, D-glutamic acid and either lysine or diaminopimelic acid (DAP). This glycan tetrapeptide repeat unit is cross-linked to adjacent glycan chains, either through a direct peptide linkage or a peptide inter-bridge (Figure 3.2). The types and numbers of cross-linking amino acids vary from organism to organism. Other unusual features of the cell wall that provide potential antimicrobial targets are DAP and the presence of two amino acids that have the D-configuration.
Bacteria can be divided into two large groups, Gram-positive and Gram-negative, on the basis of a differential staining technique called the Gram stain. Essentially, the Gram stain consists of treating a film of bacteria dried on a microscope slide with a solution of crystal violet, followed by a solution of iodine; these are then washed with an alcohol solution. In Gram-negative organisms the cells lose the crystal violet–iodine complex and are rendered colourless, whereas Gram-positive cells retain the dye. Regardless, both cell types are counter-stained with a different coloured dye, e.g. carbolfuchsin, which is red. Hence, under the light microscope Gram-negative cells appear red while Gram-positive cells are purple. These marked differences in response reflect differences in cell wall structure. The Gram-positive cell wall consists primarily of a single type of molecule whereas the Gram-negative cell wall is a multilayered structure and quite complex.
The cell walls of Gram-positive bacteria are quite thick (20–80 nm) and consist of between 60% and 80% peptidoglycan, which is extensively cross-linked in three dimensions to form a thick polymeric mesh (Figure 3.3). Gram-positive walls frequently contain acidic polysac-charides called teichoic acids; these are either ribitol phosphate or glycerol phosphate molecules that are connected by phosphodiester bridges. Because they are negatively charged, teichoic acids are partially responsible for the negative charge of the cell surface as a whole.Their function may be to effect passage of metal cations through the cell wall. In some Gram-positive bacteria glycerol-teichoic acids are bound to membrane lipids and are termed lipoteichoic acids. During an infection, lipoteichoic acid molecules released by killed bacteria trigger an inflammatory response. Cell wall proteins, if present, are generally found on the outer surface of the peptidoglycan.
The wall, or more correctly, envelope of Gram-negative cells is a far more complicated structure (Figure 3.4). Although it contains less peptidoglycan (10–20% of wall), a second membrane structure is found outside the peptidoglycan layer. This outer membrane is asymmetrical, composed of proteins, lipoproteins, phospholipids and a component unique to Gram-negative bacteria, lipopolysaccharide (LPS). Essentially, the outer membrane is attached to the peptidoglycan by a lipoprotein, one end of which is covalently attached to peptidoglycan and the other end is embedded in the outer membrane. The outer membrane is not a phospholipid bilayer although it does contain phospholipids in the inner leaf, and its outer layer is composed of LPS, a polysaccharide-lipid molecule. Proteins are also found in the outer membrane, some of which form trimers that traverse the whole membrane and in so doing form water-filled channels or porins through which small molecules can pass. Other proteins are found at either the inner or outer face of the membrane.
The LPS (Figure 3.5) is an important molecule because it determines the antigenicity of the Gram-negative cell and it is extremely toxic to animal cells. The molecule consists of three regions, namely lipid A, core polysac-charide and O-specific polysaccharide. The lipid A portion is composed of a disaccharide of glucosamine phosphate bound to fatty acids and forms the outer leaflet of the membrane. It is the lipid A component that is responsible for the toxic and pyrogenic properties of Gram-negative bacteria. Lipid A is linked to the core polysaccharide by the unique molecule ketodeoxyoctonate (KDO), and at the other end of the core is the O-polysaccharide (O-antigen), which usually contains six-carbon sugars as well as one or more unusual deoxy sugars such as abequose.
Although the outer membrane is relatively permeable to small molecules, it is not permeable to enzymes or large molecules. Indeed, one of the major functions of the outer membrane may be to keep certain enzymes that are present outside the cytoplasmic membrane from diffusing away from the cell. Moreover, the outer membrane is not readily penetrated by hydrophobic compounds and is, therefore, resistant to dissolution by detergents.
The region between the outer surface of the cytoplasmic membrane and the inner surface of the outer membrane is called the periplasm. This occupies a distance of about 12–15 nm, is gel-like in consistency and, in addition to the peptidoglycan, contains sugars and an abundance of proteins including hydrolytic enzymes and transport proteins. Table 3.2 summarizes the major differences in wall composition between Gram-positive and Gram-negative cells.
Table 3.2 Gram-positive and Gram-negative cell wall composition
Feature | Gram-positive cells | Gram-negative cells |
Peptidoglycan | 60-80% | 10-20% |
Teichoic acid | Present | Absent |
Lipoteichoic acid | Present | Absent |
Lipoprotein | Absent | Present |
Lipopolysaccharide | Absent | Present |
Protein | c.15% | c.60% |
Lipid | c.2% | c.20% |
2.2.2 Cytoplasmic membrane
Biochemically, the cytoplasmic membrane is a fragile, phospholipid bilayer with proteins distributed randomly throughout. These are involved in the various transport and enzyme functions associated with the membrane. A major difference in chemical composition between prokaryotic and eukaryotic cells is that eukaryotes have sterols in their membranes (e.g. cholesterol) whereas prokaryotes do not. The cytoplasmic membrane serves many functions, including transport of nutrients, energy generation and electron transport; it is the location for regulatory proteins and biosynthetic proteins, and it acts as a semipermeable selectivity barrier between the cytoplasm and the cell environment.
Invaginations of the cytoplasmic membrane are referred to as mesosomes. Those that form near the septum of Gram-positive cells serve as organs of attachment for the bacterial chromosome.
2.2.3 Cytoplasm
The cytoplasm consists of approximately 80% water and contains enzymes that generate ATP directly by oxidizing glucose and other carbon sources. The cytoplasm also contains some of the enzymes involved in the synthesis of peptidoglycan subunits. Ribosomes, the DNA genome (nucleoid) and inclusion granules are also found in the cytoplasm.
2.2.4 Nucleoid
The bacterial chromosome exists as a singular, covalently closed circular molecule of double-stranded DNA comprising approximately 4600 kilobase pairs. It is complexed with small amounts of proteins and RNA, but unlike eukaryotic DNA, is not associated with histones. The DNA, if linearized, would be about 1 mm in length. In order to package this amount of material the cell requires that the DNA is supercoiled into a number of domains (c. 50) and that the domains are associated with each other and stabilized by specific proteins into an aggregated mass or nucleoid. The enzymes, topoi-somerases, that control topological changes in DNA architecture are different from their eukaryotic counter-parts (which act on linear chromosomes) and therefore provide a unique biochemical target for antibiotic action.
2.2.5 Plasmids
Plasmids are relatively small, circular pieces of double-stranded extrachromosomal DNA. They are capable of autonomous replication and encode for many auxiliary functions that are not usually necessary for bacterial growth. One such function of great significance is that of antibiotic resistance (Chapter 13). Plasmids may also transfer readily from one organism to another, and between species, thereby increasing the spread of resistance.
2.2.6 Ribosomes
The cytoplasm is densely packed with ribosomes. Unlike eukaryotic cells these are not associated with a membranous structure; the endoplasmic reticulum is not a com-ponent of prokaryotic cells. Bacterial ribosomes are 70S in size, made up of two subunits of 30S and 50S. This is smaller than eukaryotic ribosomes, which are 80S in size (40S and 60S subunits). Differences will therefore exist in the size and geometry of RNA binding sites.
2.2.7 Inclusion granules
Bacteria occasionally contain inclusion granules within their cytoplasm. These consist of storage material composed of carbon, nitrogen, sulphur or phosphorus and are formed when these materials are replete in the environment to act as repositories of these nutrients when shortages occur. Examples include poly-β-hydroxy-butyrate, glycogen and polyphosphate.
2.3 Cells urface components
The surface of the bacterial cell is the portion of the organism that interacts with the external environment most directly. As a consequence, many bacteria deploy components on their surfaces in a variety of ways that allow them to withstand and survive fluctuations in the growth environment. The following sections describe a few of these components that are commonly found, although not universally, that allow bacteria to move, sense their environment, attach to surfaces and provide protection from harsh conditions.
2.3.1 Flagella
Bacterial motility is commonly provided by flagella, long (c.12μm) helical-shaped structures that project from the surface of the cell. The filament of the flagellum is built up from multiple copies of the protein flagellin. Where the filament enters the surface of the bacterium, there is a hook in the flagellum, which is attached to the cell surface by a series of complex proteins called the flagellar motor. This rotates the flagellum, causing the bacterium to move through the environment. The numbers and distribution of flagella vary with bacterial species. Some have a single, polar flagellum, whereas others are flagellate over their entire surface (peritrichous); intermediate forms also exist.
2.3.2 Fimbriae
Fimbriae are structurally similar to flagella, but are not involved in motility. Although they are straighter, more numerous and considerably thinner and shorter (3 μm) than flagella, they do consist of protein and project from the cell surface. There is strong evidence to suggest that fimbriae act primarily as adhesins, allowing organisms to attach to surfaces, including animal tissues in the case of some pathogenic bacteria, and to initiate biofilm formation. Fimbriae are also responsible for haemagglutination and cell clumping in bacteria. Among the best character-ized fimbriae are the type I fimbriae of enteric (intestinal) bacteria.
2.3.3 Pili
Pili are morphologically and chemically similar to fimbriae, but they are present in much smaller numbers (< 10) and are usually longer. They are involved in the genetic exchange process of conjugation (section 6.3).
2.3.4 Capsules and slime layers
Many bacteria secrete extracellular polysaccharides (EPS) that are associated with the exterior of the bacterial cell. The EPS is composed primarily of c. 2% carbohydrate and 98% water, and provides a gummy exterior to the cell. Morphologically, two extreme forms exist: capsules, which form a tight, fairly rigid layer closely associated with the cell, and slimes, which are loosely associated with the cell. Both forms function similarly, to offer protection against desiccation, to provide a protective barrier against the penetration of biocides, disinfectants and positively charged antibiotics, to protect against engulfment by phagocytes and protozoa and to act as a cement binding cells to each other and to the substratum in biofilms (see below). One such polymer that performs all these functions is alginate, produced by Pseudomonas aeruginosa; dextran, produced by Leuconostoc mesenteroides, is another. Both polymers may be harvested and used variously as pharmaceutical aids, surgical dressings and drug delivery systems, although the preferred source of alginate is seaweed rather than bacteria.
2.3.5 S-layers
S-layers are the most common cell wall type amongst the archaea. These consist of a two-dimensional paracrystal-line array of proteins or glycoproteins which show various ordered symmetries when viewed under the electron microscope. In many species of bacteria, S-layers are present on their outer surfaces in addition to other cell wall components such as polysaccharides. In such arrangements the S-layer is always the outermost layer. In addition to increasing the structural robustness of the cell, S-layers can act to a certain extent as an external permeability barrier.
Any surface, whether it is animate or inanimate, is of considerable importance as a microbial habitat owing to the adsorption of nutrients. A nutrient-rich microenvironment is thus produced in a nutrient-poor macroenvironment whenever a surface-liquid interface exists. Consequently, microbial numbers and activity are usually much greater on a surface than in suspension. Hence, in many natural, medical and industrial settings bacteria attach to surfaces and form multilayered communities called biofilms. These commonly contain more than one species of bacteria, which exist cooperatively together as a functional, dynamic consortium. Moreover, biofilms commonly possess unique properties that are distinct to unattached cells. Biofilm formation usually begins with pioneer cells attaching to a surface, either through the use of specific adhesins such as fimbriae, or non-specifically by EPS. Once established, these cells grow and divide to produce microcolonies, which with time, eventually coalesce to produce a biofilm. A key characteristic of biofilms is the enveloping of the attached cells in a matrix of EPS and other macromolecules. This helps to cement cells to the surface and to each other, and protects the bacteria from hazardous materials such as antibiotics and biocides, from desiccation and from engulfment by macrophages and phagocytes in much the same way as the capsules and slime layers mentioned above. In addition, strands of EPS hold the bacterial cells at a distance from one another, enabling small water channels to form in the biofilm. These channels act as a primitive circulatory system carrying trapped nutrients and oxygen to the enclosed cells and take waste products away.
Biofilms have a number of significant implications in medicine and industry. In the human body the resident cells within the biofilm are not exposed to attack by the immune system and in some instances can exacerbate the inflammatory response. An example of this is shown by the growth of Ps. aeruginosa as an alginate-enclosed biofilm in the lungs of cystic fibrosis patients. Bacterial biofilms are also profoundly less susceptible to antimicrobial agents than their free-living, planktonic counter-parts. As a consequence, bacterial biofilms that form on contaminated medical implants and prosthetic devices, manufacturing surfaces or fluid conduit systems are virtually impossible to eliminate with antibiotics or biocides. In these situations antimicrobial resistance occurs as a population or community response. Biofilms are considered in more detail in Chapter 8.
In a few bacterial genera, notably Bacillus and Clostridium, a unique process takes place in which the vegetative cell undergoes a profound biochemical change to give rise to a specialized structure called an endospore or spore (Figure 3.6). This process of sporulation is not part of a reproductive cycle, but the spore is a highly resistant cell that enables the producing organism to survive in adverse environmental conditions such as lack of moisture or essential nutrients, or exposure to toxic chemicals, radiation or high temperatures. Because of their extreme resistance to radiation, ethylene oxide and heat, all sterilization processes for pharmaceutical products have been designed to destroy the bacterial spore (Chapter 21). Removal of the environmental stress may lead to germi-nation of the spore back to the vegetative cell form.
4.1 Endospore structure
Endospores are differentiated cells that possess a grossly different structure to that of the parent vegetative cell in which they are formed. The structure of the spore is much more complex than that of the vegetative cell in that it has many layers surrounding a central core (Figure 3.7). The outermost layer is the exosporium composed of protein; within this are the spore coats, which are also proteinaceous but with a high cysteine content, the cortex that consists of loosely cross-linked peptidoglycan and the central core that contains the genome. Characteristic of the spore is the presence of dipicolinic acid and high levels of calcium ions which complex together. The core is also partially dehydrated, containing only 10–30% of the water content of the vegetative cells. Dehydration has been shown to increase resistance to both heat and chem-icals. In addition, the pH of the core is about 1 unit lower than the cytoplasm of the vegetative cell and contains high levels of core-specific proteins that bind tightly to the DNA and protect it from potential damage. These core-specific proteins also function as an energy source for the outgrowth or germination of a new vegetative cell from the endospore.
< div class='tao-gold-member'>
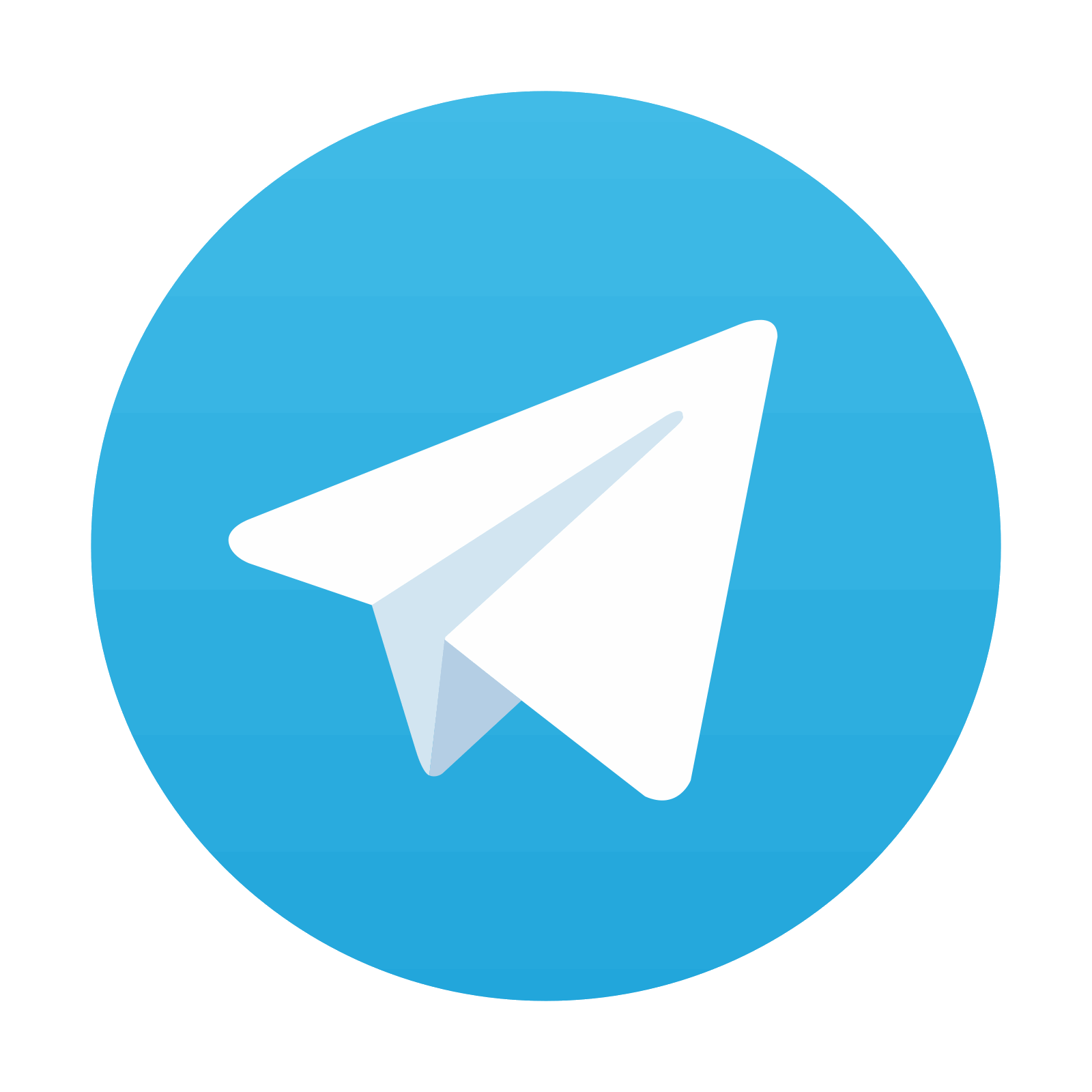