Genetic predisposition
Down syndrome
Fanconi anemia
Other inherited bone marrow failure syndromes:
Shwachman-Diamond
Diamond-Blackfan
Dyskeratosis congenita
Kostmann’s
Familial platelet disorder (RUNX1 mutation)
DNA repair defects, e.g., Bloom syndrome
Other germline mutations e.g., involving CEBPA, DDX41, GATA2
Prior hematologic disorder
Chronic myeloid leukemia
Myeloproliferative neoplasms
Myelodysplastic syndrome
Paroxysmal nocturnal hemoglobinuria
Exposure to environmental or therapeutic agents
Chronic exposure to benzene and derivatives
Ionizing radiation
Chemotherapeutic agents:
Alkylating agents
Topoisomerase Il-targeting drugs
Molecular Basis of Disease
Considerable progress has been made in understanding the cytogenetic and molecular basis of AML over the course of the last four decades. This has had a major impact on the laboratory workup of patients with suspected AML, with definition of the karyotype and mutational profile of the leukemic cells being of critical importance by serving to identify biologically distinct subsets of disease and predicting likely response to therapy and overall survival. Age of presentation has an important bearing on disease features, with balanced chromosomal translocations being relatively common in children and younger adults, while AML in older patients is characterized by a more common picture of whole chromosome losses (monosomy) and gains (e.g., trisomy) and losses (deletion) or gains (duplication, unbalanced translocation) of chromosomal segments that often occur in the context of a complex karyotype.
Cytogenetic Classification of AML
Balanced Chromosomal Rearrangements
Improved understanding of the molecular basis of AML was incorporated into the 2008 revision of the World Health Organization (WHO) Classification of Tumours of Haematopoietic and Lymphoid Tissues [1, 2], which necessitates application of a range of laboratory tests including cytogenetics and molecular testing to complement morphological and immunophenotypic assessment for disease diagnosis and categorization. The WHO classification is organized in a hierarchical fashion, with the first group being AML with particular balanced translocations or inversions (and their molecular counterparts), which are defined as “AML with recurrent genetic abnormalities” (Table 40.2). These include t(15;17)(q22;q12~21), the diagnostic hallmark of acute promyelocytic leukemia (APL), which accounts for approximately 12 % of AML cases. Cloning of the translocation breakpoints in the early 1990s [3–5] showed that t(15;17) leads to fusion of the gene that encodes the myeloid transcription factor, retinoic acid receptor alpha (RARA), with a previously unknown gene designated PML (for promyelocytic leukemia), which has subsequently been found to be involved in growth suppression and regulation of apoptosis [6]. While the vast majority of APL cases have an underlying PML–RARA fusion, in approximately 1–2 % RARA is fused to an alternative partner [7] and classified as “AML with a variant RARA translocation.” These rare subtypes of APL include involvement of ZBTB16 (PLZF), NPM1, NUMA, FIP1L1, and BCOR, as a result of the t(11;17)(q23;q21), t(5;17)(q35;q21), t(11;17)(q13;q21), t(4;17)(q12;q21), and t(X;17)(p11;q21), respectively, and PRKAR1A and STAT5B in rearrangements involving 17q [8–14]. The nature of the fusion partner has an important bearing on disease biology, particularly the response to molecularly targeted therapies, i.e., all transretinoic acid (ATRA) and arsenic trioxide (ATO) [15]. ATRA targets the ligand-binding domain of the RARα moiety in the C-terminal region of the fusion proteins (as well as wild-type RARα). Sensitivity to ATRA has been documented in APL subtypes involving PML, NPM1, NUMA, and FIP1L1 [15], whereas PLZF-RARα and STAT5B-RARα have both been associated with primary resistance to retinoids and a poorer prognosis [14, 16, 17]. To date, sensitivity to ATO has only been demonstrated in PML–RARA-positive APL, reflecting the capacity of ATO to bind directly to the PML moiety of the fusion protein inducing its degradation via the proteasome [18]. Therefore, ATO should not be used for the treatment of APL as part of front-line therapy or in the context of suspected relapse, unless positive for PML–RARA.
Table 40.2
Cytogenetic abnormalities used in the WHO classification of AML
Cytogenetic abnormalities used to define entities within the WHO category: “AML with recurrent genetic abnormalities” | |
t(8;21)(q22;q22); RUNX1-RUNX1T1 inv(16)(p13.1q22) or t(16;16)(p13.1;q22); CBFB-MYH11 t(15;17)(q22;q12); PML-RARA t(9;11)(p22;q23); MLLT3-MLL | t(6;9)(p23;q34); DEK-NUP214 inv(3)(q21q26.2) or t(3;3)(q21;q26.2); GATA2-EVI1b t(1;22)(p13;q13); RBM15-MKL1 |
Cytogenetic abnormalities sufficient to diagnose WHO category: “AML with myelodysplasia-related changes” Complex karyotype | |
Defined as three or more unrelated abnormalities, none of which can be a translocation or inversion associated with “AML with recurrent genetic abnormalities” | |
Unbalanced abnormalities –7 or del(7q) –5 or del(5q) i(17q) or t(17p) –13 or del(13q) del(11q) del(12p) or t(12p) del(9q) idic(X)(q13) | Balanced abnormalities t(11;16)(q23;p13.3)a t(3;21)(q26.2;q22.1)a t(1;3)(p36.3;q21.1) t(2;11)(p21;q23)a t(5;12)(q33;p12) t(5;7)(q33;q11.2) t(5;17)(q33;p13) t(5;10)(q33;q21) t(3;5)(q25;q34) |
Approximately 10 % of patients with AML are classified as having core-binding factor (CBF) leukemia with balanced chromosomal rearrangements that disrupt genes that encode components of the heterodimeric transcription factor complex, comprising RUNX1 (AML1, CBFα) and CBFβ, which plays a critical role in hematopoiesis [19]. The CBFα subunit is targeted by the t(8;21)(q22;q22), which fuses RUNX1 (AML1, CBFA2) to the gene encoding the RUNX1T1 (formerly ETO for eight twenty one) transcriptional repressor, thereby potentially silencing RUNX1 target genes [19, 20]. The β-subunit is targeted by the inv(16)(p13.1q22) or the less common t(16;16)(p13.1;q22), in which CBFB is fused to the gene encoding myosin heavy chain (MYH11). RUNX1 is a recurrent translocation target in non-CBF acute leukemias, with fusions to ETV6 (TEL) as a result of the cytogenetically cryptic t(12;21)(p13;q22) in pediatric acute lymphoblastic leukemia (ALL), or to a range of partners in AML [21, 22], including MECOM (EVI1/MDS1) as a result of t(3;21)(q26;q22) in “AML with myelodysplasia-related changes” (Table 40.2). RUNX1 also has been implicated in therapy-related leukemias arising following exposure to drugs targeting topoisomerase II [23, 24].
The MLL (for myeloid/lymphoid or mixed-lineage leukemia, at present named KMT2A) gene located at 11q23 is a further recurrent translocation target in acute leukemia, with almost 80 partner genes now characterized [25]. MLL is an epigenetic regulator that plays a critical role in hematopoiesis, modulating HOX gene expression [26]. The most common MLL translocation observed in AML is the t(9;11)(p22;q23), which occurs in approximately 2 % of cases and leads to fusion of MLL with MLLT3 (formerly known as AF9). AML with t(9;11)(p22;q23) has been associated with a relatively favorable outcome in some pediatric [27] and adult [28] AML studies and is distinguished as a separate entity in the 2008 WHO classification. Apart from the t(9;11), the most frequent other MLL translocations observed in AML are t(6;11)(q27;q23) involving MLLT4 (AF6), t(11;19)(q23;p13.3) involving MLLT1 (ENL), t(11;19)(q23;p13.1) involving ELL, and complex rearrangements between 10p12 and 11q23 (e.g., a reciprocal translocation and an inversion of an 11q segment translocated to 10p12 or inverted insertion of an 11q segment into 10p12 or a 10p segment into 11q23), in which the fusion partner is MLLT10 (AF10) [29, 30]. While translocations involving MLL are observed in de novo leukemia and account for the majority of leukemias presenting in infancy, the locus is also a recurrent translocation target in therapy-related leukemias [31], particularly those arising following exposure to the epipodophyllotoxin class of topoisomerase II inhibitors [32]. Such cases are classified within the WHO as “therapy-related AML.”
The remaining subtypes of AML distinguished as separate disease entities on the basis of cytogenetics are those characterized by the t(6;9)(p23;q34), inv(3)(q21q26.2) or t(3;3)(q21;q26.2), and t(1;22)(p13;q13). The t(6;9)(p23;q34) is found in approximately 1 % of AML and leads to fusion of DEK at 6p23 with NUP214 (CAN) at 9q34, which encodes a component of the nuclear pore complex [33, 34]. The inv(3) or t(3;3) occurs in a similar proportion of AML cases and is associated with upregulation of the zinc finger transcription factor MECOM (EVI1), which is involved in normal hematopoiesis. AML with inv(3) or t(3;3) may present de novo, or secondary to prior myelodysplastic syndrome (MDS), and is characterized by a normal or elevated presenting platelet count and abnormal megakaryopoiesis with micromegakaryocytes in the bone marrow (BM) [35]. MECOM has long been implicated in leukemogenesis, having been identified as a recurrent integration target in murine retroviral mutagenesis screens [36, 37]; further evidence has been provided by characterization of MDS associated with insertional activation of MECOM occurring as a complication of gene therapy for chronic granulomatous disease [38]. Interestingly, these have provided important insights into potential mechanisms underlying acquisition of additional cytogenetic abnormalities in AML, with both cases showing monosomy 7, which is well recognized as a frequent secondary abnormality in cases with inv(3) or t(3;3). Forced overexpression of MECOM was found to disrupt normal centrosome duplication, suggesting that activation of MECOM as a result of retroviral insertion or chromosomal translocation leads to genomic instability, giving rise to acquisition of additional changes such as monosomy 7 involved in progression to MDS and AML.
AML with t(1;22)(p13;q13) is extremely rare (only approximately 40 cases reported worldwide [39]) and is associated with acute megakaryoblastic leukemia occurring in infants and young children (<3 years of age), particularly those without Down syndrome. The translocation fuses RBM15 (for RNA-binding motif protein 15, also known as OTT) with MKL1 (for MegaKaryoblastic Leukemia [Translocation] 1, or MAL) [40, 41], which is involved in normal megakaryocyte maturation [42].
AML with Myelodysplasia-Related Cytogenetic Changes
Apart from distinguishing AML with recurrent genetic abnormalities (as described above) from cases lacking one of these aberrations (or their molecular counterparts), cytogenetics is also used in the 2008 WHO classification as a criterion (in conjunction with ≥20 % leukemic blasts in the bone marrow [BM]) to define a subgroup of “AML with myelodysplasia-related changes” (Table 40.2). Some of these cytogenetic entities are relatively common, such as monosomy 5 and 7 (−5 and −7) or deletion of the long arms of these chromosomes [del(5q) and del(7q)], which often occur as part of a complex karyotype. Noteworthy is the observation that while −7 is recurrently present both as a sole chromosome aberration and as part of a complex karyotype, −5 is very rare in patients with noncomplex karyotypes [43]. Moreover, most patients with a complex karyotype, with −5 detected using banding techniques (e.g., G-banding), do not harbor true monosomy 5, because segments from a seemingly missing chromosome 5 can be found using spectral karyotyping [44] or fluorescence in situ hybridization (FISH) [45] in marker chromosomes or unbalanced structural aberrations only partially identified by G-banding. While balanced translocations such as t(11;16)(q23;p13.3) involving MLL, t(3;21)(q26.2;q22.1) involving RUNX1, and t(3;5)(q25;q34) that generates the NPM1–MLF1 fusion [46] may be initiating lesions in the development of leukemia, many of the changes considered as “myelodysplasia related” entail loss of chromosomal segments or whole chromosomes, which are poorly understood at the molecular level and may represent secondary and cooperating lesions in AML pathogenesis. Some of the aberrations designated “myelodysplasia related,” especially balanced translocations and idic(X)(q13), are extremely rare [47], making characterization of their biological features and establishment of their impact on the clinical outcome challenging.
Mutations Involved in the Pathogenesis of AML
While balanced chromosomal rearrangements are considered to be primary lesions involved in the pathogenesis of AML, they are insufficient to mediate the full leukemic phenotype, requiring acquisition of additional cooperating mutations. Work is currently in progress to define the spectrum of such mutations in cytogenetically defined subsets of AML (Table 40.3), particularly using high-throughput sequencing technologies. In addition, a major focus of research in recent years has been the deciphering of the molecular events underlying the pathogenesis of AML with normal karyotype (CN-AML). Such strategies also carry the potential of generating important targets for molecular approaches to disease therapy.
Table 40.3
Genes and their recurrent mutations in AML, with associated clinical characteristics and outcomes
Gene symbol a /chromosome location | Gene name | Overall mutation frequency | Mutation frequency in CN-AML | Mutation frequency in other cytogenetic subsets | Associations of mutations with clinical and molecular characteristics | Clinical significance of the presence of mutations |
---|---|---|---|---|---|---|
FLT3 (CD135, STK1, FLK2)/13q12 | Fms-related tyrosine kinase 3 | FLT3 – ITD: FLT3 – TKD: | FLT3 – ITD: FLT3 – TKD: <60 years 9 % [56] 12 % [51] | FLT3 – ITD: t(6;9): 90 % [54] inv(3)/t(3;3): 9 % [54] +8: 7–23 % [51] del(7q): 4 % [51] −5/del(5q): 3 % [54] FLT3- TKD: del(5q): 9 % [51] −7: 5 % [51] del(7q): 9 % [51] | Pts with FLT3 -ITD have worse DFS, EFS, and OS than those with no FLT3-ITD, but FLT3-ITD does not impact significantly on the CR achievement probability [53, 54] Outcome is particularly poor for pts with high FLT3-ITD mutant-to-FLT3-WT allelic ratio [54, 57, 58] | |
CEBPA/19q13.1 | CCAAT/enhancer-binding protein (C/EBP) alpha | 3 % single mutations [59] 4 % double mutations [59] | MRC favorable – 0 % mutation [59] MRC intermediate – 3 % single mutations – 5 % double mutations [59] MRC adverse – 3 % single mutations – 0 double mutations [59] | Among all AML pts, double CEBPA mutations are associated with better OS and RFS than those of pts with single CEBPA mutations and CEBPA-WT. On MVA, double CEBPA mutations have been an independent favorable prognostic factor for OS, RFS, and CIR. Double CEBPA mutations bestow longer OS also among pts in the MRC intermediate cytogenetic-risk group [59] In CN-AML pts, OS of pts with double CEBPA mutations was longer than OS of CEBPA-WT and single CEBPA-mutated pts. On MVA, double CEBPA mutations were an independent favorable factor for OS [62] In younger (<60 years) CN-AML pts, both single and double CEBPA mutations conferred longer OS than OS of CEBPA-WT pts, but only double CEBPA mutations retained favorable prognostic significance in MVA for OS, EFS, and RFS [60] | ||
NPM1 (NPM, B23)/5q35.1 | Nucleophosmin (nucleolar phospho-protein B23, numatrin) | −5/del(5q): 3 % [67] t(11;v)(q23;v): 6 % [66] | NPM1 mutations are more frequent in women, associated with higher WBC, % of BM blasts and platelet counts; low or absent CD34 and high CD33 expression on blasts. NPM1 mutated pts twice as often have FLT3-ITD and FLT3-TKD than NPM1-WT pts. NPM1 mutations almost never coexist with double CEBPA mutations, MLL-PTD, R172 IDH2, and BCOR mutations [63–67] | In younger adults (<60 years), NPM1 mutations confer superior CR rates, EFS, DFS, and OS in the absence of FLT3-ITD [52, 64–67] In older pts (≥60 years), NPM1 mutations alone are an independent favorable prognostic factor [68] | ||
IDH1/2q32-qter | Isocitrate dehydrogenase 1 (NADP+), soluble | CBF AML: 1.5 % [70] t(15;17): 1 % [69] | Among all AML pts, IDH1 mutations are associated with higher platelet counts, higher % of BM blasts [69], FAB M1 BM morphology [70], and CN-AML [69, 70] | |||
IDH2/15q21-qter | Isocitrate dehydrogenase 2 (NADP+), mitochondrial | Among all AML pts, IDH2 mutations are associated with older age, MRC cytogenetic intermediate-risk group and NPM1 mutations [72]. Pts with IDH2 mutations involving residue R172 have lower WBC and an abnormal, MRC intermediate-risk karyotype more often than pts with IDH2 mutations involving R140, most of whom have CN-AML IDH2 mutations involving R140 are associated with FAB M1 BM morphology [73] | Among all AML pts, IDH2 mutations involving R172 are associated with low CR rates and increased CIR IDH2 mutations involving R140 constitute an independent favorable prognostic factor for relapse risk and OS [72] | |||
TET2 (FLJ20032)/4q24 | Tet methylcytosine dioxygenase 2 | 7 % in younger pts (≤60 years) [74] 24 % in older pts (>60 years) [74] | 15 % in younger pts (<60 years) [77] 29 % in older pts (≥60 years) [77] | +8: 35 % [74] | TET2 mutations have no impact on outcome of all AML pts [75] | |
DNMT3A/2p23 | DNA (cytosine-5-)-methyltransferase 3 alpha | 18 % in younger pts (<60 years) [79] | 34 % overall [80] 33 % in older pts (≥60 years) [80] | CBF AML: 1 % [79] | Among all AML pts, DNMT3A mutations are associated with older age, higher WBC, % of BM blasts and platelet counts, CN-AML, FLT3-ITD, NPM1, and IDH1 mutations, and higher MLL5 expression [79] In CN-AML, DNMT3A mutations are associated with higher WBC and % of BM blasts and are found more often in pts with NPM1 mutations, FLT3-ITD and CEBPA-WT [80] | In all AML pts, DNMT3A mutations independently predict a shorter OS, but are not associated with RFS or CR rate [79] DNMT3A mutations are associated with shorter OS and RFS in all pts and an intermediate cytogenetic-risk subset without FLT3-ITD and NPM1 mutations [78] In CN-AML overall, DNMT3A mutations are associated with shorter DFS (but not OS [80]), with lower CR rates and shorter OS on MVA [79] and shorter EFS and OS, which remain significant on MVA [81] In CN-AML pts <60 years, non-R882-DNMT3A mutations confer shorter DFS and OS [80] In CN-AML pts ≥60 years, R882-DNMT3A mutations are associated with shorter DFS and OS [80] |
ASXL1 (KIAA0978)/20q11 | Additional sex combs like transcriptional regulator 1 | 3 % in younger pts (<60 years) [85] 16 % in older pts (≥60 years) [85] | sole +8: 32 % [82] | Among all pts, ASXL1 mutations are associated with lower WBC [83], FAB M1 BM morphology, expression of HLA-DR and CD34 [82] and very rarely coexist with NPM1 mutations and FLT3-ITD [83, 84] In CN-AML pts, ASXL1 mutations are associated with lower WBC, % of BM and blood blasts, CEBPA mutations and low incidence of NPM1 mutations and FLT3-ITD [85] | In all AML pts, ASXL1 mutations confer lower CR rates and worse OS in univariable analyses and worse OS in MVA [83] In CN-AML pts ≥60 years, ASXL1 mutations confer lower CR rates and worse DFS, OS, and EFS [85] | |
BCOR (FLJ20285, KIAA1575)/Xp11.4 | BCL6 corepressor | 2.5 % [86] | 4 % [86] | None found among 131 pts with abnormal karyotypes [86] | In CN-AML, BCOR mutations very rarely coexist with NPM1 mutations and FLT3-ITD; ~45 % of BCOR-mutated pts harbor DNMT3A and RUNX1 mutations [86] | In CN-AML, BCOR mutations are associated with a shorter OS and EFS [86] |
BCORL1 (FLJ11362)/Xq25-q26.1 | BCL6 corepressor-like 1 | 6 % [87] | 2 % [87] | NA | Prognostic significance is not yet established | |
PHF6/Xq26 | PHD finger protein 6 | NA | NA | PHF6 mutations are seven times more common in men than women; associated with FAB M0, M1, and M2 BM morphology, detected mostly in pts with CN-AML and +8 [88] | Prognostic significance is not yet established | |
MLL (ALL-1, CXXC7, HRX, HTRX1, TRX1, MLL1A, KMT2A)/11q23 | Myeloid/ lymphoid or mixed-lineage leukemia (trithorax homologue, Drosophila) | sole +11: 91 % [93] | In younger (<60 years) CN-AML pts, MLL-PTD is associated with lower WBC and less extramedullary involvement and negatively associated with NPM1 mutations [52, 94] and mutually exclusive with CEBPA mutations [52] In older (≥60 years) CN-AML pts, MLL-PTD is associated with lower hemoglobin and incidence of NPM1 mutations and absence of CEBPA and R172 IDH2 mutations [95] | |||
RUNX1 (AML1, CBFA2, AMLCR1, PEBP2A2)/21q22.3 | Runt-related transcription factor 1 | 5 % [49] 6 % in younger pts (<60 years) [96] | 16 % in older pts (≥60 years) [98] | t(15;17): 0 % [96] t(8;21): 0 % [96] inv(16): 0 % [96] +13: 90 % [97] +8: 30 % [97] +11: 31 % [97] +21: 36 % [97] −7/del(7q): 29 % [97] | Among all pts, RUNX1 mutations are associated with FAB M0 and M2 BM morphology, MLL-PTD and IDH1–IDH2 mutations and wild-type NPM1 and CEBPA [96] In CN-AML, RUNX1 mutations are associated with older age, lower hemoglobin, WBC and % blood blasts, ASXL1 mutations, and wild-type NPM1 and CEBPA [98] | Among all pts, RUNX1 mutations associated with resistant disease and worse RFS, OS, and EFS [96, 97] |
KIT (PBT, C-Kit, CD117, SCFR)/4q11-q12 | v-kit Hardy-Zucker-man 4 feline sarcoma viral oncogene homologue | 6 % [49] 2 % (only KIT D816 mutations) [50] | ||||
TP53 (p53, LFS1)/17p13.1 | Tumor protein p53 | 1 % [104] | t(8;21): 7 % [104] t(15;17): 0 % [104] inv(16): 0 % [104] t(11;v)(q23;v) : 0 % [104] +13 sole: 10 % [104] +8 sole: 7 % [104] −7 sole: 0 % [104] | TP53 mutation and loss are associated with older age and larger number of chromosome aberrations in CK pts [105] | TP53 mutations and loss confer lower CR rates and worse EFS, RFS, and OS in pts with a complex karyotype [105] | |
WT1 (GUD, AWT1, WAGR, WIT-2)/11p13 | Wilms tumor 1 | 7 % in older pts (≥60 years) [110] | In CN-AML pts, WT1 mutations confer: worse DFS and OS (but not CR probability) [109] no impact on RFS or OS [108] CN-AML pts with WT1 mutations and FLT3-ITD have lower CR rates and worse RFS and OS than pts with WT1 mutations without FLT3-ITD [108] | |||
NRAS (N-ras)/1p13.2 | Neuroblastoma RAS viral (v-ras) oncogene homologue | t(3;5): 63 % [112] +8: 17 % [112] −7/del(7q): 8 % [112] | No significant associations, except for lower WBC in one study [113] | RAS mutations reported to increase sensitivity of AML blasts to higher doses of cytarabine [114] | ||
KRAS (KRAS1, KRAS2)/12p12.1 | v-Ki-ras2 Kirsten rat sarcoma viral oncogene homologue | 4 % [112] | inv(16): 17 % [112] t(8;21): 5 % [112] t(15;17): 4 % [112] inv(3): 10 % [112] t(3;5): 0 % [112] t(11;v)(q23;v): 6 % [112] +8: 3 % [112] −7/del(7q): 7 % [112] CK: 3 % [112] | No significant associations [112] | No significant impact on outcome [112] | |
CBL (CBL2, c-Cbl, RNF55)/11q23.3-qter | Cbl proto-oncogene, E3 ubiquitin protein ligase | 0 % [115] | inv(16): 5 % [115] t(8;21): 5 % [115] abn(11q): 15 % [115] | Mutations are detected mainly in pts with inv(16), t(8;21), and abn(11q) [115] | Prognostic significance is not yet established | |
Genes of the cohesion complex: STAG1 (SA-1, SCC3A)/3q22.2-q22.3 STAG2 (SA, SCC3B)/Xq25 SMC3 (CSPG6, BAM, HCAP, SMC3L1)/10q25 RAD21 (hHR21, KIAA0078, SCC1)/8q24.11 SMC1A (SMC1, SMC1L1, DXS423E, KIAA0178, SB1.8, Smcb)/Xp11.22-p11.21 | Stromal antigen 1 Stromal antigen 2 Structural maintenance of chromosomes 3 RAD21 homologue (S. pombe) Structural maintenance of chromosomes 1A | 0–1.8 % [118] | 8 % [118] | t(15;17): 0 % [119] | No significant impact on CR rates, RFS or OS in all AML pts, nor in all CN-AML pts, CN-AML pts with mutated NPM1, or CN-AML pts with mutated NPM1 and wild-type FLT3 [118] | |
Spliceosomal genes SF3B1 (Hsh155, Prp10, PRPF10, SAP155, SF3b155)/2q33.1 SRSF2 (SFRS2, PR264, SC-35, SC35, SFRS2A)/17q25.2 U2AF1 (U2AFBP, RN, RNU2AF1, U2AF35)/21q22.3 | Splicing factor 3b, subunit 1, 155 kDa Serine/arginine-rich splicing factor 2 U2 small nuclear RNA auxiliary factor 1 | 6 % in adult de novo AML [121] 0 % in pediatric AML [121] 28 % in AML evolved from MPN [122] 41 % in AML with 20–30 % BM blasts [120] | +13: 88 % [123] | SRSF2 mutations conferred worse OS in AML evolved from MPN [122] | ||
GATA2 (NFE1B)/3q21 | GATA binding protein 2 | In CN-AML pts, mutations in GATA2 are found in 39 % of pts with double CEBPA mutations, but in no pts with monoallelic CEBPA mutations or CEBPA-WT [126] | Among pts with double CEBPA mutations, GATA2 mutations had no significant impact on EFS or OS of CN-AML pts [126] and on EFS or OS of intermediate-risk AML pts [124] Among intermediate-risk AML pts with double CEBPA mutations and no FLT3-ITD, GATA2 mutations had no significant impact on CIR and OS [125] |
Fms-Related Tyrosine Kinase 3 (FLT3)
The FLT3 tyrosine kinase has been an intense focus for research. Groups working in Japan in the late 1990s identified two major classes of receptor-activating mutations of FLT3 that, cumulatively, are found in approximately 30 % of AML patients [127, 128]. In normal hematopoiesis, the membrane-spanning FLT3 receptor is expressed on early progenitor cells including CD34+ hematopoietic stem cells and plays an important role in proliferation, survival, and differentiation. High levels of abnormal FLT3 expression are seen in 70–100 % of AML [129].
FLT3 internal tandem duplication mutations (FLT3-ITDs) are in-frame palindromic duplications of exons 14 and 15 of the FLT3 gene, between three and 400 bp in length, that disrupt the autoinhibitory function of the FLT3 juxtamembrane domain, leading to activation of signaling pathways downstream of FLT3. Pooled data show an overall FLT3-ITD incidence of 23 % in newly diagnosed AML, with a lower incidence in children [130]. Although ITD length has previously been thought not to influence prognosis, some longer ITDs, which integrate within the first tyrosine kinase domain of the receptor, are associated with lower complete remission (CR) rate and poor relapse-free survival [131]. FLT3-ITDs are accompanied by NPM1 mutations in a significant proportion of CN-AML patients (see below) [58]. FLT3-ITDs are predominantly seen in CN-AML, being associated with proliferative disease with a high presenting white blood cell (WBC) count and poor prognosis in terms of significantly increased relapse rate and decreased survival in both adults and children [48, 132]. FLT3-ITD mutations at diagnosis are highly heterogeneous with respect to FLT3-ITD allelic ratio, ITD length, and cooperating partner mutations. Patients with a high “ITD mutant-to-wild-type FLT3 allelic ratio,” sometimes due to mitotic recombination leading to partial uniparental disomy, have an especially poor prognosis and usually relapse within 12 months of initial treatment [57]. Relapsed FLT3-ITD-mutated AML carries a particularly dismal prognosis; blasts in this setting are highly dependent on FLT3 kinase signaling, carrying a particularly high FLT3-mutant allelic burden with the wild-type FLT3 allele often virtually absent [133].
A further 7–10 % of AML patients have mutations involving the activation loop within the tyrosine kinase domain of the FLT3 receptor (FLT3-TKDs), usually single base substitutions (most frequently D835Y) or small deletions. Although similarly FLT3 activating and associated with high presenting WBC levels, prognostic implications of FLT3-TKDs are less clear; patient series describe conflicting adverse, intermediate, and even favorable prognostic associations [51, 56, 134]. In contrast to FLT3-ITD mutations, FLT3-TKD mutations appear to frequently be “late genetic hits,” are often lost at relapse, and have subtly different effects than ITDs on FLT3 downstream signaling [135].
Whether or not allogeneic stem cell transplantation (SCT) in first CR improves outcomes in FLT3-ITD AML patients remains controversial [136], although the presence of the mutation in the context of wild-type NPM1 is considered an indication for SCT in the consensus document by the European LeukemiaNet [137]. The high incidence and clear deleterious prognostic impact of FLT3-ITD mutations, however, coupled with the tangible clinical gains achieved through targeting dysregulated tyrosine kinase activity in other malignancies, such as chronic myeloid leukemia (CML) and breast cancer, have provided a strong rationale for the development of FLT3-targeted therapy in AML. Several multi-kinase inhibitors with FLT3-inhibitory activity, the most developed of which being midostaurin (PKC412), lestaurtinib (CEP701), and sorafenib, have been assessed in combination with chemotherapy in international phase III clinical trials, with results currently awaited. Newer and more potent FLT3-inhibitory molecules, most notably quizartinib (AC220) and ASP2215, are in earlier stages of clinical development [138].
Other molecular abnormalities that have been consistently shown to confer a poorer prognosis include mutations in the RUNX1 gene [96–98, 139] and partial tandem duplications of the MLL gene (MLL-PTD) [52, 90–92], although recent data indicate that intensive consolidation therapy that includes autologous transplant in first CR may improve the outcome of CN-AML patients with the latter rearrangement [94].
CEBPA and NPM1
Major steps forward in understanding the molecular pathogenesis of AML were the discoveries of mutations in the genes encoding CCAAT/enhancer-binding protein-α (CEBPA) and nucleophosmin (NPM1) that serve to identify subsets of patients with relatively favorable prognosis [63, 140] and which were recognized as provisional disease entities in the 2008 WHO classification [1]. Mutations in CEBPA, which encodes a myeloid transcription factor, were first described in 2001 and occur in approximately 10 % of cytogenically normal AML (CN-AML) [141]. Mutations cluster in both the amino- and carboxy-terminal regions, with the former leading to expression of a truncated 30 kDa isoform of CEBPA (p30) and loss of the 42 kDa full-length protein (p42) [142]. Carboxy-terminal mutations affect regions involved in mediating dimerization and DNA binding. Interestingly, in the majority of patients with CEBPA mutations, both alleles are involved, combining an upstream mutation in one allele with a downstream mutation in the other [141, 142]. The analysis of patient samples has shown that CEBPA mutations can be inherited, with progression to AML in later life being associated with acquisition of additional mutations, which can include involvement of the other CEBPA allele [143, 144]. Significant insights into the biology of CEBPA mutations have been provided by murine models, which have shown how loss of p42 expression (mimicking biallelic N-terminal CEBPA mutations), or compound heterozygous mutations affecting amino- and carboxy-terminal regions in combination, affects hematopoiesis and gives rise to AML [145, 146]. While early studies reported that CEBPA mutation predicts a relatively favorable outcome in AML [52, 147–149], subsequent studies have shown that the more favorable prognosis is seen in the subset of patients with biallelic mutations, especially those who lack FLT3-ITD [59, 60, 150–152].
Mutations in NPM1, discovered by Brunangelo Falini and colleagues in 2005 [63], represent the most common molecular lesion identified in AML to date, occurring in a third of cases, including 50–60 % of those with CN-AML. Over 30 different mutations have been described, which involve the C-terminal region of the protein. These lead to loss of tryptophan residues and generation of a nuclear export signal resulting in delocalization of nucleophosmin from the nucleoli to the cytoplasm [153]. NPM1 mutation is considered, an AML-defining lesion, being stable in the vast majority of cases over the disease course [154, 155]. Indeed, NPM1 mutation has been shown to enhance self-renewal of hematopoietic progenitors, associated with expanded myelopoiesis leading to the development of AML in a murine model [156]. Patients with AML harboring an NPM1 mutation in the absence of a FLT3-ITD have a relatively favorable prognosis [64–66]. NPM1 mutations also have been shown to be strong, independent predictors of better outcome in older patients, especially those aged 70 years and older [68]. Whereas most studies have focused on CN-AML [157], patients with AML with cytogenetic abnormalities that would be considered to have a standard risk but who harbor an NPM1 mutation also have a better outcome in the absence of FLT3-ITD; however, those with FLT3-ITD in the absence of the protective effect of an NPM1 mutation have a very poor prognosis [43, 158]. Whether the combined FLT3-ITD/NPM1-mutated genotype is itself associated with an intermediate or adverse outcome remains open to debate.
Mutations Identified in Epigenetic Modifiers
Using a variety of high-throughput technologies, recurring mutations that affect regulation of gene expression, directly or through more indirect mechanisms, have been identified in recent years. In a landmark study, genome sequencing was undertaken to identify the presence of biologically relevant mutations in a case of NPM1-mutated CN-AML [159]. A mutation was identified in codon 132 of isocitrate dehydrogenase 1 (IDH1), which was already known to be involved in the pathogenesis of gliomas, with IDH1 mutations predicting a relatively favorable prognosis in this group of solid tumors [160]. In the cytoplasm, IDH1 catalyzes the conversion of isocitrate to α-ketoglutarate, generating NADPH. The IDH1 mutation that affects the arginine amino acid at codon 132 (R132) reduces α-ketoglutarate formation, but in addition alters enzyme function leading to generation of 2-hydroxyglutarate (2-HG), associated with increased reactive oxygen species (ROS) levels, HIF1α induction, and upregulation of VEGF [161, 162]. IDH1 mutations at R132 occur in approximately 7 % of AML, particularly in those with a normal karyotype and NPM1 mutation (reviewed by Löwenberg [163]). Based upon these findings, AMLs were screened for mutations in the mitochondrial homologue IDH2, which were identified in an additional 10 % of AML cases. The IDH2 mutations cluster at codons R140 (8 %) and R172 (2 %) [69, 71, 163, 164]; these mutations are prognostically distinct, with the latter predicting a poor prognosis [71–73]. Interestingly, an inverse relationship has been observed between mutations occurring in the IDH genes and TET2 [74–77, 165]. The latter was found to be a recurrent mutation target in a range of myeloid neoplasms including AML by array-based comparative genomic hybridization (aCGH) and single nucleotide polymorphism (SNP) profiling. TET2 has subsequently been shown to be involved in the regulation of hydroxylation of 5-methylcytosine, with disruption of TET2 leading to myeloid transformation in murine models [166, 167]. Methylation profiles of IDH– and TET2-mutated AMLs were found to be highly comparable, with evidence to suggest that accumulation of 2-HG secondary to IDH mutation inhibits TET2 function [165]. Moreover, it has recently been shown that TET2 binding to DNA is regulated by WT1, providing an explanation why WT1 mutations are also mutually exclusive of TET2 and IDH mutations [233, 234]. Consequently, this pathway is deregulated in a third of AML cases.
Studies examining the clinical significance of TET2 mutations have yielded somewhat conflicting results. Gaidzik et al. [75] found no impact of TET2 mutations on outcome of a cytogenetically heterogeneous patient population, and this was also true for the whole cohort of CN-AML patients analyzed by Metzeler et al. [77]. However, another study determined that TET2 mutations confer worse outcome in AML patients with intermediate-risk cytogenetic findings (defined using the Southwest Oncology Group [SWOG] criteria) [74], and two studies demonstrated adverse impact of TET2 mutations on the outcome of those CN-AML patients who are classified in the Favorable Genetic Group of the European LeukemiaNet (ELN) classification (i.e., CN-AML with mutated CEBPA and/or mutated NPM1 without FLT3-ITD) [76, 77].
Re-sequencing of the index NPM1-mutated CN-AML case with IDH1 mutation originally characterized by Mardis and colleagues [159] identified an additional acquired alteration in another gene involved in epigenetic regulation, namely, the DNA methyltransferase DNMT3A [168]. This gene was confirmed to be mutated in approximately 20 % of all AML patients and in approximately 30 % of those with a normal karyotype [79–81, 168–171]. Mutations in DNMT3A are associated with the presence of NPM1 mutation and FLT3-ITD and are distributed throughout the gene, with a mutational hotspot at position R882. The presence of a DNMT3A mutation predicts a poorer outcome (Table 40.3).
High-throughput technologies have identified mutations in additional epigenetic modifiers in AML. These include ASXL1 (additional sex combs like transcriptional regulator 1), which is involved in the regulation of histone methylation [82, 83, 85, 172], with mutations occurring 3–5 times more often in patients aged 60 years or older [83, 85] than in younger patients and being associated with the presence of RUNX1 or CEBPA mutations, the absence of NPM1 and FLT3-ITD mutations, and poorer clinical outcome [85]. Using exome sequencing, mutations in genes located on the X-chromosome encoding the related transcriptional repressors BCOR and BCORL1 have been identified [86, 87]. The former is a rare translocation target in APL [12]; is mutated in approximately 4 % of CN-AML, detected in cases with wild-type NPM1; and may also confer a poorer prognosis [86]. There are few data on BCORL1 mutations, which were first identified in older adults with secondary AML and subsequently found to be mutated in 6 % of a cohort of unselected AML patients [87]. In addition, approximately 3 % of AML have mutations in the PHF6 gene, which encodes a homeodomain protein and is frequently mutated in T-cell ALL [49, 88].
Indications for Testing
Thorough laboratory investigation of AML is fundamental to the optimal clinical management of the disease and is not only critical to establish the diagnosis but also to identify patients who may benefit from targeted therapies, to inform risk stratification and to guide post-remission therapy including the need for stem cell transplantation. Prognostic factors in AML can be subdivided into those defined at diagnosis (pretreatment) or following the start of antileukemic therapy (posttreatment) (Table 40.4). With the exception of age, patient performance status, and type of AML (de novo vs secondary), pretreatment determination of the likely outcome to therapy is dependent on laboratory investigation, with karyotype, NPM1, CEBPA, and FLT3 mutation status being the most important factors identified to date. Predicting the likely outcome following initiation of therapy falls entirely within the realm of the laboratory and, for patients achieving morphological complete remission (CR), is dependent on the detection of minimal residual disease (MRD), for which flow cytometry and molecular diagnostic strategies have been developed.
Table 40.4
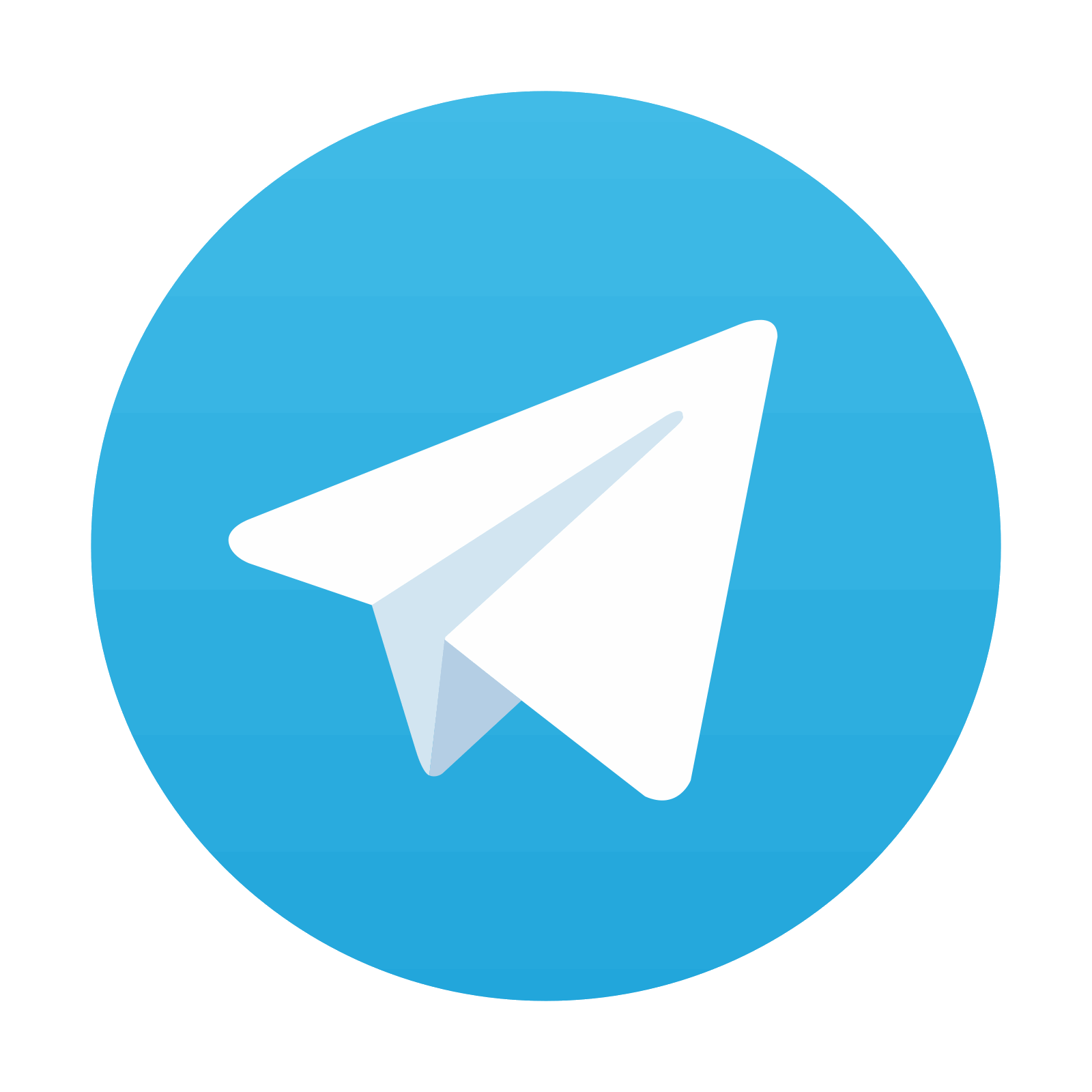
Prognostic factors for relapse in patients with newly diagnosed AML
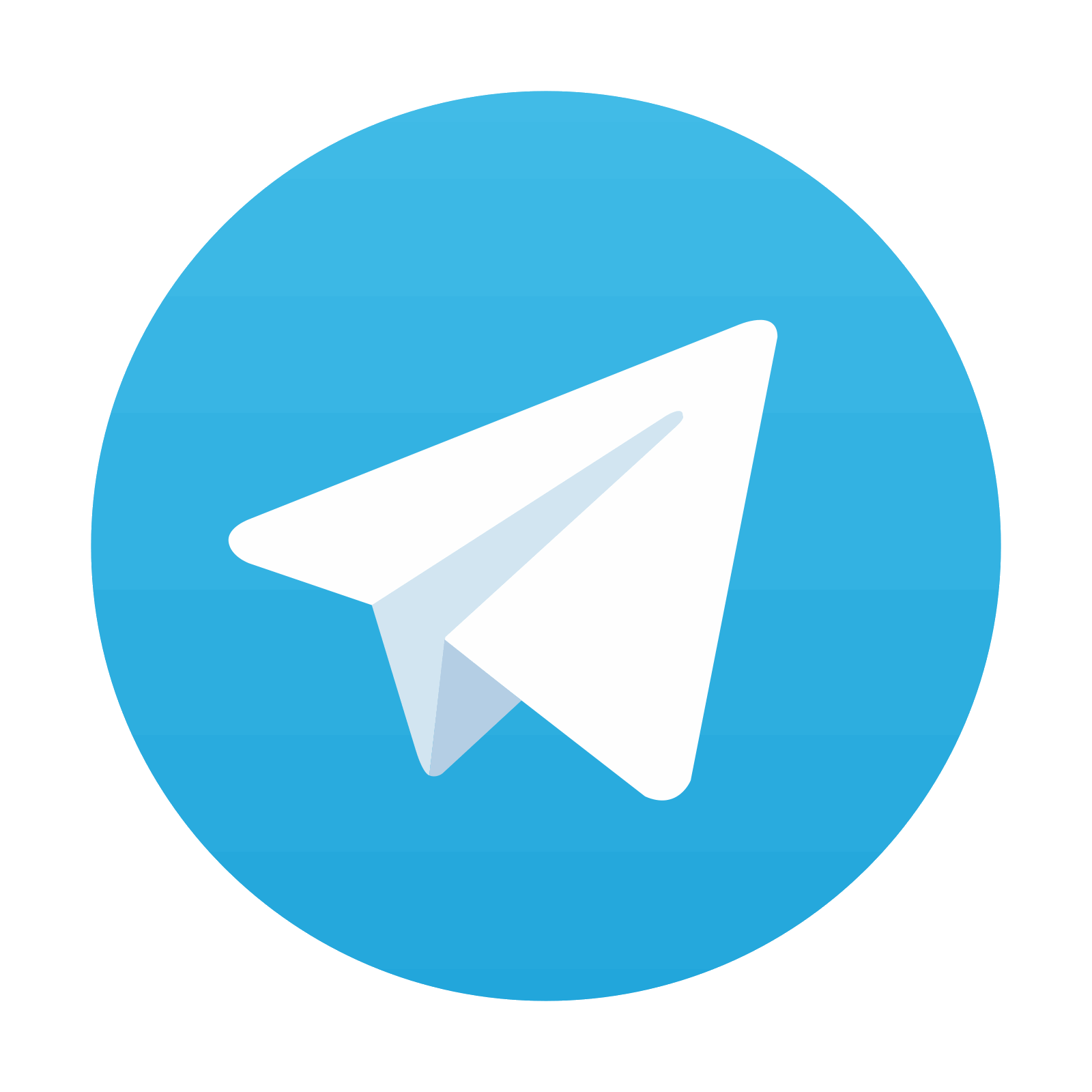
Stay updated, free articles. Join our Telegram channel
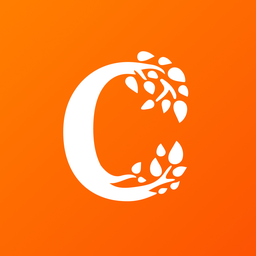
Full access? Get Clinical Tree
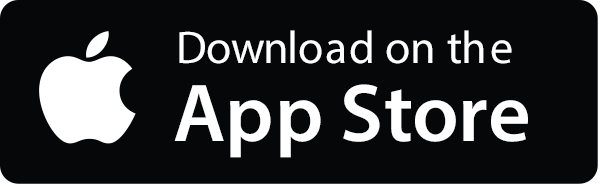
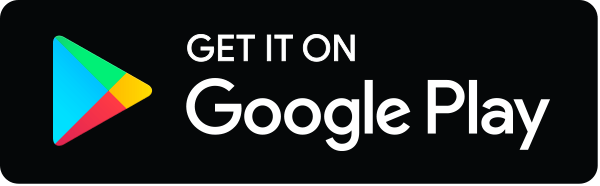
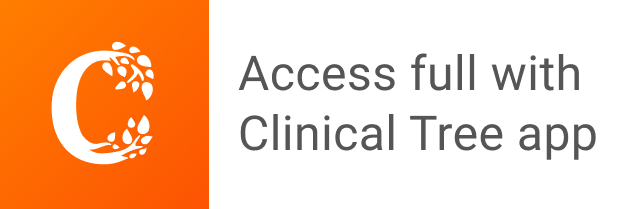