Proteins are produced by the process of translation, which occurs on ribosomes and is directed by messenger RNA (mRNA). The genetic message encoded in DNA is first transcribed into mRNA, and the nucleotide sequence in the coding region of the mRNA is then translated into the amino acid sequence of the protein.
Translation of the Code. The portion of mRNA that specifies the amino acid sequence of the protein is read in codons, which are sets of three nucleotides that specify individual amino acids (Fig. 14.1). The codons on mRNA are read sequentially in the 5′-to-3′ direction, starting with the 5′-AUG (or “start” codon) that specifies methionine and sets the reading frame and ending with a 3′-termination (or “stop”) codon (UAG, UGA, or UAA). The protein is synthesized from its N-terminus to its C-terminus.
FIGURE 14.1. Binding of transfer RNA (tRNA) to a codon on messenger RNA (mRNA). The tRNA contains an amino acid at its 3′-end that corresponds to the codon on mRNA with which the anticodon of the tRNA can base-pair. Note that the codon–anticodon pairing is complementary and antiparallel.
Each amino acid is carried to the ribosome by an aminoacyl-transfer RNA (tRNA) (i.e., a tRNA with an amino acid covalently attached). Base pairing between the anticodon of the tRNA and the codon on the mRNA ensures that each amino acid is inserted into the growing polypeptide at the appropriate position.
Synthesis of the Protein. Initiation involves the formation of a complex containing the initial methionyl-tRNA bound to the AUG “start” codon of the mRNA and the “P” site of the ribosome. It requires guanosine triphosphate (GTP) and proteins known as eukaryotic initiation factors (eIFs).
Elongation of the polypeptide involves three steps: (1) binding of an aminoacyl-tRNA to the “A” site on the ribosome, where it base-pairs with the second codon on the mRNA; (2) formation of a peptide bond between the first and second amino acids; and (3) translocation, movement of the mRNA relative to the ribosome, so that the third mRNA codon moves into the “A” site. These three elongation steps are repeated until a termination codon aligns with the site on the ribosome where the next aminoacyl-tRNA would normally bind. Release factors bind instead, causing the completed protein to be released from the ribosome.
After one ribosome binds and moves along the mRNA, translating the polypeptide, another ribosome can bind and begin translation. The complex of a single mRNA with multiple ribosomes is known as a polysome.
Folding and Modification and Targeting of the Protein. Folding of the polypeptide into its three-dimensional configuration occurs as the polypeptide is being translated. This process involves proteins called chaperones. Modification of amino acid residues in a protein occurs during or after translation. Proteins synthesized on cytosolic ribosomes are released into the cytosol or transported into mitochondria, peroxisomes, and the nucleus. Proteins synthesized on ribosomes attached to the rough endoplasmic reticulum (RER) are destined for lysosomes, cell membranes, or secretion from the cell. These proteins are transferred to the Golgi complex, where they are modified and targeted to their ultimate locations.
THE WAITING ROOM 
Lisa N., a 4-year-old patient with β+-thalassemia intermedia (see Chapter 13), showed no improvement in her symptoms at her second visit. Her hemoglobin level was 7.3 g/dL (reference range for females, 12 to 16 g/dL).
Jay S. is a 9-month-old male infant of Ashkenazi Jewish parentage. His growth and development were normal until age 5 months, when he began to exhibit mild, generalized muscle weakness. By 7 months, he had poor head control, slowed development of motor skills, and was increasingly inattentive to his surroundings. His parents also noted unusual eye movements and staring episodes. On careful examination of his retinae, his pediatrician observed a “cherry red” spot within a pale macula. The physician suspected Tay–Sachs disease and sent samples of his whole blood to the molecular biology–genetics laboratory.
Paul T. returned to his physician’s office after 5 days of azithromycin therapy (see Chapter 11) feeling significantly better. The sputum sample from his previous visit had been cultured. The results confirmed that his respiratory infection was caused by Streptococcus pneumoniae and that the organism was sensitive to penicillin, macrolides (e.g., erythromycin, clarithromycin), tetracycline, and other antibiotics.
Edna R., a 25-year-old junior medical student, brings her healthy 4-month-old daughter, Beverly, to the pediatrician for her second diphtheria, pertussis, and tetanus (DPT, acellular pertussis) immunization, along with immunizations for pneumococcal, inactivated polio, haemophilus influenzae, and rotavirus. Edna R. tells the doctor that her great great aunt had died of diphtheria during an epidemic many years ago.
I. The Genetic Code
Transcription, the transfer of the genetic message from DNA to RNA, and translation, the transfer of the genetic message from the nucleotide language of nucleic acids to the amino acid language of proteins, both depend on base pairing. In the late 1950s and early 1960s, molecular biologists attempting to decipher the process of translation recognized two problems. The first involved decoding the relationship between the language of the nucleic acids and the language of the proteins, and the second involved determining the molecular mechanism by which translation between these two languages occurs.
Twenty different amino acids are commonly incorporated into proteins, and therefore the protein alphabet has 20 characters. The nucleic acid alphabet, however, has only four characters, corresponding to the four nucleotides of mRNA (A, G, C, and U). If two nucleotides constituted the code for an amino acid, then only 42 or 16 amino acids could be specified. Therefore, the number of nucleotides that code for an amino acid has to be at least three, providing 43 or 64 possible combinations or codons, more than required, but not excessive.
Scientists set out to determine the specific codons for each amino acid. In 1961, Marshall Nirenberg produced the first crack in the genetic code (the collection of codons that specifies all the amino acids found in proteins). He showed that poly(U), a polynucleotide in which all the bases are uracil, produced polyphenylalanine in a cell-free protein-synthesizing system. Thus, UUU must be the codon for phenylalanine. As a result of experiments using synthetic polynucleotides in place of mRNA, other codons were identified.
The pioneering molecular biologists recognized that, because amino acids cannot bind directly to the sets of three nucleotides that form their codons, adapters are required. The adapters were found to be tRNA molecules. Each tRNA molecule contains an anticodon and covalently binds a specific amino acid at its 3′-end (see Chapters 11 and 13). The anticodon of a tRNA molecule is a set of three nucleotides that can interact with a codon on mRNA (see Fig. 14.1). In order to interact, the codon and anticodon must be complementary (i.e., they must be able to form base pairs in an antiparallel orientation). Thus, the anticodon of a tRNA serves as the link between an mRNA codon and the amino acid that the codon specifies.
Obviously, each codon present within mRNA must correspond to a specific amino acid. Nirenberg found that trinucleotides of known base sequence could bind to ribosomes and induce the binding of specific aminoacyl-tRNAs (i.e., tRNAs with amino acids attached covalently). As a result of these and the earlier experiments, the relationship between all 64 codons and the amino acids they specify (the entire genetic code) was determined by the mid-1960s (Table 14.1).
Three of the 64 possible codons (UGA, UAG, and UAA) terminate protein synthesis and are known as stop or nonsense codons. The remaining 61 codons specify amino acids. Two amino acids each have only one codon (AUG for methionine; UGG for tryptophan). The remaining amino acids have multiple codons.
A. The Code Is Degenerate Yet Unambiguous
Because many amino acids are specified by more than one codon, the genetic code is described as degenerate, which means that an amino acid may have more than one codon. However, each codon specifies only one amino acid, and the genetic code is thus unambiguous.
Inspection of a codon table shows that in most instances of multiple codons for a single amino acid, the variation occurs in the third base of the codon (see Table 14.1). Crick noted that the pairing between the 3′-base of the codon and the 5′-base of the anticodon does not always follow the strict base-pairing rules that he and Watson had previously discovered (i.e., A pairs with U, and G with C). This observation resulted in the wobble hypothesis.
At the third base of the codon (the 3′-position of the codon and the 5′-position of the anticodon), the base pairs can wobble. For example, G can pair with U, and A, C, or U can pair with the unusual base hypoxanthine (I) found in tRNA. Thus, three of the four codons for alanine (GCU, GCC, and GCA) can pair with a single tRNA that contains the anticodon 5′-IGC-3′ (Fig. 14.2). If each of the 61 codons for amino acids required a distinct tRNA, cells would contain 61 tRNAs. However, because of wobble between the codon and anticodon, fewer than 61 tRNAs are required to translate the genetic code.
FIGURE 14.2. Base pairing of codons for alanine with 5′-IGC-3′. A. The variation is in the third base. B. The first three of these codons can pair with a transfer RNA (tRNA) that contains the anticodon 5′-IGC-3′. Hypoxanthine (I) is an unusual base found in tRNA that can form base pairs with U, C, or A. It is formed by the deamination of adenine. Hypoxanthine is the base attached to ribose in the nucleoside inosine. The single-letter abbreviation for hypoxanthine is I, referring to the nucleoside inosine.
All organisms studied so far use the same genetic code, with some rare exceptions. One exception occurs in human mitochondrial mRNA, in which UGA codes for tryptophan instead of serving as a stop codon, AUA codes for methionine instead of isoleucine, and CUA codes for threonine instead of leucine.
B. The Code Is Nonoverlapping
mRNA does not contain extra nucleotides, or punctuation, to separate one codon from the next, and the codons do not overlap. Each nucleotide is read only once. Beginning with a start codon (AUG) near the 5′-end of the mRNA, the codons are read sequentially, ending with a stop codon (UGA, UAG, or UAA) near the 3′-end of the mRNA.
C. Relationship between mRNA and the Protein Product
The start codon (AUG) sets the reading frame, the order in which the sequence of bases in the mRNA is sorted into codons (Fig. 14.3). The order of the codons in the mRNA determines the sequence in which amino acids are added to the growing polypeptide chain. Thus, the order of the codons in the mRNA determines the linear sequence of amino acids in the protein.
FIGURE 14.3. Reading frame of messenger RNA (mRNA). A. For any given mRNA sequence, there are three possible reading frames (1, 2, and 3). B. An AUG near the 5′-end of the mRNA (the start codon) sets the reading frame for the translation of a protein from the mRNA. The codons are read in linear order, starting with this AUG. (The other potential reading frames are not used. They would give proteins with different amino acid sequences.)
II. Effects of Mutations
Mutations that result from damage to the nucleotides of DNA molecules or from unrepaired errors during replication (see Chapter 12) can be transcribed into mRNA and therefore can result in the translation of a protein with an abnormal amino acid sequence. Various types of mutations can occur, which have different effects on the encoded protein (Table 14.2).
TYPE | DESCRIPTION | EXAMPLE |
Point | A single base change | |
Silent | A change that specifies the same amino acid | CGA → CGG |
Arg → Arg | ||
Missense | A change that specifies a different amino acid | CGA → CCA |
Arg → Pro | ||
Nonsense | A change that produces a stop codon | CGA → UGA |
Arg → Stop | ||
Insertion | An addition of one or more bases | |
Deletion | A loss of one or more bases |
A. Point Mutations
Point mutations occur when only one base in DNA is altered, producing a change in a single base of an mRNA codon. There are three basic types of point mutations: silent mutations, missense mutations, and nonsense mutations. Point mutations are said to be “silent” when they do not affect the amino acid sequence of the protein. For example, a codon change from CGA to CGG does not affect the protein because both of these codons specify arginine (see Table 14.1). In missense mutations, one amino acid in the protein is replaced by a different amino acid. For example, a change from CGA to CCA causes arginine to be replaced by proline. A nonsense mutation causes the premature termination of a polypeptide chain. For example, a codon change from CGA to UGA causes a codon for arginine to be replaced by a stop codon, so the synthesis of the mutant protein terminates at this point.
B. Insertions, Deletions, and Frameshift Mutations
An insertion occurs when one or more nucleotides are added to DNA. If the insertion does not generate a stop codon, a protein with more amino acids than normal could be produced.
When one or more nucleotides are removed from DNA, the mutation is known as a deletion. If the deletion does not affect the normal start and stop codons, a protein with fewer than the normal number of amino acids could be produced.
A frameshift mutation occurs when the number of inserted or deleted nucleotides is not a multiple of three (Fig. 14.4). The reading frame shifts at the point where the insertion or deletion begins. Beyond that point, the amino acid sequence of the protein translated from the mRNA differs from the normal protein.
FIGURE 14.4. A frameshift mutation. The insertion of a single nucleotide (the A in the dotted red box) causes the reading frame to shift so that the amino acid sequence of the protein translated from the mRNA is different after the point of insertion. A similar effect can result from the insertion or deletion of nucleotides if the number inserted or deleted is not a multiple of three.
III. Formation of Aminoacyl-tRNA
A tRNA that contains an amino acid attached covalently to its 3′-end is called an aminoacyl-tRNA and is considered to be charged. Aminoacyl-tRNAs are named both for the amino acid and the tRNA that carries the amino acid. For example, the tRNA for alanine (tRNAAla) acquires alanine to become alanyl-tRNAAla. A particular tRNA recognizes only the AUG start codon that initiates protein synthesis and not other AUG codons that specify insertion of methionine within the polypeptide chain. This initiator methionyl-tRNAMet is denoted by the subscript “i” in methionyl-tRNAiMet.
Amino acids are attached to their tRNAs by highly specific enzymes known as aminoacyl-tRNA synthetases. Twenty different synthetases exist, one for each amino acid. Each synthetase recognizes a particular amino acid and all of the tRNAs that carry that amino acid.
The formation of the ester bond that links the amino acid to the tRNA by an aminoacyl-tRNA synthetase is an energy-requiring process that occurs in two steps. The amino acid is activated in the first step when its carboxyl group reacts with adenosine triphosphate (ATP) to form an enzyme-aminoacyl–adenosine monophosphate (AMP) complex and pyrophosphate (Fig. 14.5). The cleavage of a high-energy bond of ATP in this reaction provides energy, and the subsequent cleavage of pyrophosphate by a pyrophosphatase helps to drive the reaction by removing one of the products. In the second step, the activated amino acid is transferred to the 2′- or 3′-hydroxyl group (depending on the type of aminoacyl-tRNA synthetase that catalyzes the reaction) of the ribose connected to the 3′-terminal A residue of the tRNA, and AMP is released (recall that all tRNAs have a CCA added to their 3′-end posttranscriptionally). The energy in the aminoacyl-tRNA ester bond is subsequently used in the formation of a peptide bond during the process of protein synthesis. The aminoacyl-tRNA synthetase provides the first error-checking step in preserving the fidelity of translation. The enzymes check their work, and if the incorrect amino acid has been linked to a particular tRNA, the enzyme will remove the amino acid from the tRNA, and try again utilizing the correct amino acid.
FIGURE 14.5. Formation of aminoacyl-tRNA. The amino acid is first activated by reacting with ATP. The amino acid is then transferred from the aminoacyl-AMP to tRNA. AMP, adenosine monophosphate; ATP, adenosine triphosphate; Pi, inorganic phosphate; PPi, pyrophosphate; tRNA, transfer RNA.
Some aminoacyl-tRNA synthetases use the anticodon of the tRNA as a recognition site as they attach the amino acid to the hydroxyl group at the 3′-end of the tRNA (Fig. 14.6). However, other synthetases do not use the anticodon but recognize only bases located at other positions in the tRNA. Nevertheless, insertion of the amino acid into a growing polypeptide chain depends solely on the bases of the anticodon, through complementary base pairing with the mRNA codon.
FIGURE 14.6. Some aminoacyl-tRNA synthetase recognition sites on tRNA. Each aminoacyl-tRNA synthetase is specific for certain tRNA, which it “recognizes” by binding the sequences of nucleotides called recognition sites, shown in green. In some cases the anticodon is a recognition site, in others it is not. This is true for human tRNAs as well as for those shown here. E. coli, Escherichia coli; tRNA, transfer RNA.
IV. Process of Translation
Translation of a protein involves three steps: initiation, elongation, and termination. It begins with the formation of the initiation complex. Subsequently, synthesis of the polypeptide occurs by a series of elongation steps that are repeated as each amino acid is added to the growing chain (Fig. 14.7). Termination occurs where the mRNA contains an in-frame stop codon, and the completed polypeptide chain is released.
FIGURE 14.7. Overview of the process of translation. (1) Once a transfer RNA (tRNA) has donated its amino acid to the growing polypeptide chain (which itself is still linked to a tRNA), it is released from the mRNA. (2) A new aminoacyl-tRNA binds to the correct codon in the mRNA to donate its amino acid to the growing polypeptide chain.
A. Initiation of Translation
In eukaryotes, initiation of translation involves the formation of an initiation complex composed of methionyl-tRNAiMet, mRNA, and a ribosome (Fig. 14.8). Methionyl-tRNAiMet (also known as Met-tRNAiMet) initially forms a complex with the protein eIF2, which binds GTP. This complex then binds to the small (40S) ribosomal subunit with the participation of eIF3. eIF3 also participates in preventing the premature association of the 60S ribosomal subunit with the pre initiation complex. The cap at the 5′-end of the mRNA binds to components of the eIF4 complex, eIF4F, known as the cap-binding complex. eIF4F is a complex comprising eIF4E, eIF4A, and eIF4G. The mRNA, in association with the cap-binding complex, then binds to the eIFs-Met-tRNAiMet–40S ribosome complex. In a reaction that requires hydrolysis of ATP (because of the helicase activity of an eIF subunit), this complex unwinds a hairpin loop in the mRNA and scans the mRNA until it locates the AUG start codon (usually the first AUG in the mRNA). GTP is hydrolyzed, the initiation factors (IFs) are released, and the large ribosomal (60S) subunit binds. The ribosome is now complete. It contains one small and one large subunit, and it has three binding sites for tRNA, known as the P (peptidyl), A (aminoacyl), and E (ejection) sites. During initiation, Met-tRNAiMet binds to the ribosome at the P site, which is located initially at the start codon for translation. Eukaryotes also contain a Kozak consensus sequence which is recognized by the ribosome as the translational start site (the sequence is A or G – CCAUGG, where the purine base is three bases upstream of the AUG start codon). The Kozak sequence aids in defining the initial AUG codon for translation. Loss of this sequence reduces the efficiency of translational initiation.
FIGURE 14.8. Initiation of protein synthesis. This is a simplified version of translational initiation as many more initiation factors and steps are required. A site, aminoacyl site on the ribosome; ADP, adenosine diphosphate; ATP, adenosine triphosphate; E site, free transfer RNA (tRNA) ejection site (the A, P, and E sites or portions of them are indicated by dashed lines); eIF, eukaryotic initiation factor; GDP, guanosine diphosphate; GTP, guanosine triphosphate; Met, methionyl; mRNA, messenger RNA; Pi, inorganic phosphate; P site, peptidyl site on the ribosome.
The initiation process differs for prokaryotes and eukaryotes (Table 14.3). In bacteria, the initiating methionyl-tRNA is formylated, producing a formyl-methionyl-tRNAfMet that participates in the formation of the initiation complex (Fig. 14.9). Only three IFs are required to generate this complex in prokaryotes, compared with the dozen or more required by eukaryotes. The ribosomes also differ in size. Prokaryotes have 70S ribosomes, composed of 30S and 50S subunits, and eukaryotes have 80S ribosomes, composed of 40S and 60S subunits. Unlike eukaryotic mRNA, bacterial mRNA is not capped. Identification of the initiating AUG triplet in prokaryotes occurs when a sequence in the mRNA (known as the Shine–Dalgarno sequence) binds to a complementary sequence near the 3′-end of the 16S ribosomal RNA (rRNA) of the small ribosomal subunit.
TABLE 14.3 Differences between Eukaryotes and Prokaryotes in the Initiation of Protein Synthesis
EUKARYOTES | PROKARYOTES | |
Binding of mRNA to small ribosomal subunit | Cap at 5′-end of mRNA binds eIFs and 40S ribosomal subunit containing tRNAiMet. mRNA is scanned for AUG start codon within the Kozak consensus sequence | Shine–Dalgarno sequence upstream of initiating AUG binds to complementary sequence in 16S rRNA |
First amino acid | Methionine | Formyl-methionine |
Initiation factors | eIFs (12 or more) | IFs (3) |
Ribosomes | 80S | 70S |
(40S and 60S subunits) | (30S and 50S subunits) |
eIF, eukaryotic initiation factor; IF, initiation factor; mRNA, messenger RNA; rRNA, ribosomal RNA; tRNAiMet, initiator methionyl-tRNA.
FIGURE 14.9. Bacterial tRNA containing formyl-methionine. The initial methionine is not formylated in eukaryotic protein synthesis. tRNA, transfer RNA.
Initiation of translation is also regulated at the level of the IFs. For example, insulin, an anabolic hormone, stimulates general protein synthesis by activating the initiation factor eIF4E. Normally, eIF4E is bound to an inhibitor protein, designated 4E-binding protein (4E-BP). When insulin binds to its cell surface receptor, it initiates an intracellular sequence of events resulting in phosphorylation of 4E-BP. Phosphorylated 4E-BP no longer binds to eIF4E, and eIF4E is now free to participate in the initiation of protein synthesis.
Similarly, eIF2 is a regulator of the initiation step in protein synthesis. When it is phosphorylated, it is inactive, and protein synthesis cannot begin. Conditions such as starvation, heat shock, and viral infection result in phosphorylation of eIF2 by a specific kinase.
The regulation of globin synthesis by heme in reticulocytes illustrates the role of eIF2 in the regulation of translation. Reticulocytes, which are the precursors of red blood cells, synthesize the oxygen-carrying hemoglobin molecules from the globin polypeptide chains and the Fe-binding pigment, heme. In the absence of heme, the rate of initiation of globin synthesis decreases. Heme acts by inhibiting the phosphorylation of the initiation factor eIF2. Thus, eIF2 is active in the presence of heme, and globin synthesis is initiated.
And finally, both eIF2 and elongation factor 1 (EF1) are types of heterotrimeric G proteins (see Chapter 10). They dramatically change their conformation and actively form complexes when they bind GTP but become inactive and dissociate when they hydrolyze this GTP to guanosine diphosphate (GDP). GTP can then displace the bound GDP to reactivate the eIF2 or the EF1.
B. Elongation of Polypeptide Chains
After the initiation complex is formed, addition of each amino acid to the growing polypeptide chain involves binding of an aminoacyl-tRNA to the A site on the ribosome, formation of a peptide bond, and translocation of the peptidyl-tRNA to the P site (Fig. 14.10). The peptidyl-tRNA contains the growing polypeptide chain.
FIGURE 14.10. Elongation of a polypeptide chain. (1) Binding of valyl-tRNAVal to the A site. (2) Formation of a peptide bond. (3) Translocation. (4) Ejection of the free tRNA. After step 4, step 1 is repeated using the aminoacyl-tRNA for the new codon in the A site. Steps 2, 3, and 4 follow. These four steps keep repeating until termination occurs. A site, aminoacyl site; EF, elongation factor; E site, free transfer RNA ejection site; GDP, guanosine diphosphate; GTP, guanosine triphosphate; mRNA, messenger RNA; Pi, inorganic phosphate; P site, peptidyl site; tRNA, transfer RNA.
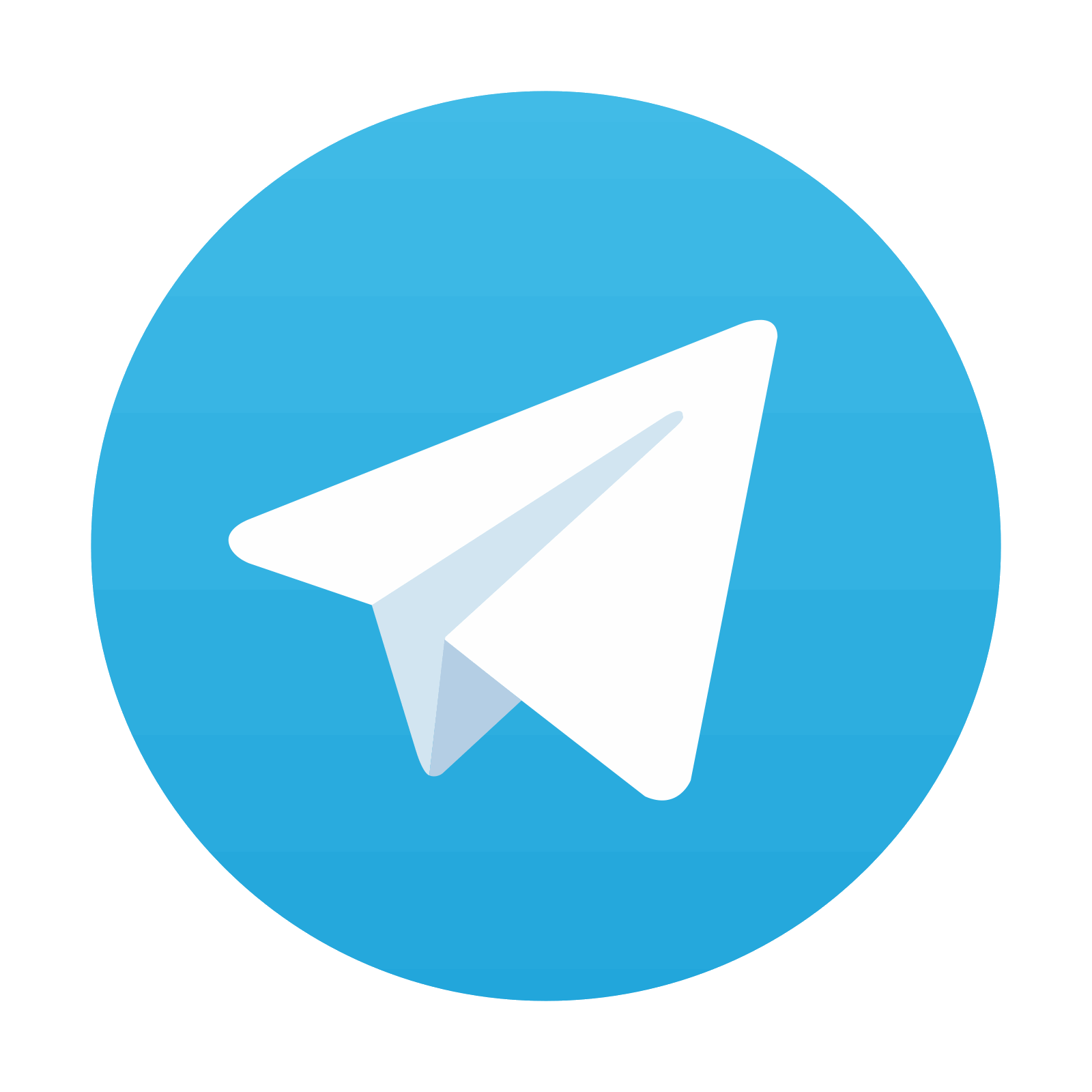
Stay updated, free articles. Join our Telegram channel
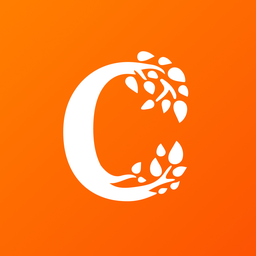
Full access? Get Clinical Tree
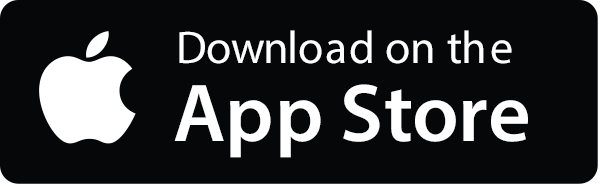
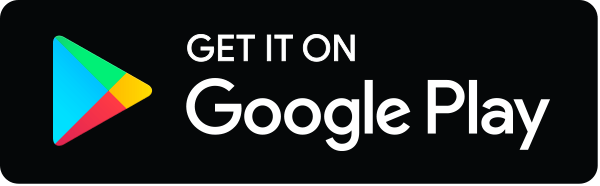