The tricarboxylic acid cycle (TCA cycle) accounts for more than two-thirds of the adenosine triphosphate (ATP) generated from fuel oxidation. The pathways for oxidation of fatty acids, glucose, amino acids, acetate, and ketone bodies all generate acetyl coenzyme A (acetyl CoA), which is the substrate for the TCA cycle. As the activated two-carbon acetyl group is oxidized to two molecules of CO2, energy is conserved as NADH, FAD(2H), and guanosine triphosphate (GTP) (Fig. 23.1). NADH and FAD(2H) subsequently donate electrons to O2 via the electron transport chain, with the generation of ATP from oxidative phosphorylation. Thus, the TCA cycle is central to energy generation from cellular respiration.
FIGURE 23.1 Summary of the TCA cycle. The major pathways of fuel oxidation generate acetyl CoA, which is the substrate for the TCA cycle. The number of carbons in each intermediate of the cycle is indicated in parentheses by the name of the compound. Acetyl CoA, acetyl coenzyme A; CoASH, coenzyme A; FAD, flavin adenine dinucleotide; GDP, guanosine diphosphate; GTP, guanosine triphosphate; NAD, nicotinamide adenine dinucleotide; Pi, inorganic phosphate; TCA, tricarboxylic acid.
Within the TCA cycle, the oxidative decarboxylation of α-ketoglutarate is catalyzed by the multisubunit α-ketoglutarate dehydrogenase complex, which contains the coenzymes thiamin pyrophosphate, lipoate, and FAD. A similar complex, the pyruvate dehydrogenase complex (PDC), catalyzes the oxidation of pyruvate to acetyl CoA, thereby providing a link between the pathways of glycolysis and the TCA cycle (see Fig. 23.1).
The two-carbon acetyl group is the ultimate source of the electrons that are transferred to NAD+ and FAD and also the carbon in the two CO2 molecules that are produced. Oxaloacetate is used and regenerated in each turn of the cycle (see Fig. 23.1). However, when cells use intermediates of the TCA cycle for biosynthetic reactions, the carbons of oxaloacetate must be replaced by anaplerotic (filling-up) reactions, such as the pyruvate carboxylase reaction.
The TCA cycle occurs in the mitochondrion, where its flux is tightly coordinated with the rate of the electron transport chain and oxidative phosphorylation through feedback regulation that reflects the demand for ATP. The rate of the TCA cycle is increased when ATP utilization in the cell is increased through the response of several enzymes to adenosine diphosphate (ADP) levels, the NADH/NAD+ ratio, the rate of FAD(2H) oxidation, or the Ca2+ concentration. For example, isocitrate dehydrogenase is allosterically activated by ADP.
There are two general consequences to impaired functioning of the TCA cycle: (1) an inability to generate ATP from fuel oxidation and (2) an accumulation of TCA cycle precursors. For example, inhibition of pyruvate oxidation in the TCA cycle results in its reduction to lactate, which can cause lactic acidosis. The most common situation leading to an impaired function of the TCA cycle is a relative lack of oxygen to accept electrons in the electron transport chain.
THE WAITING ROOM 
Otto S., a 26-year-old medical student, has faithfully followed his diet and aerobic exercise program of daily tennis and jogging (see Chapter 20). He has lost a total of 33 lb and is just 23 lb from his college weight of 154 lb. His exercise capacity has markedly improved; he can run for a longer time at a faster pace before noting shortness of breath or palpitations of his heart. Even his test scores in his medical school classes have improved.
Ann R. suffers from anorexia nervosa (see Chapters 1, 3, and 9). In addition to a low body weight, decreased muscle mass, glycogen, and fat stores, she has iron-deficiency anemia (see Chapter 15). She has started to gain weight and is trying a daily exercise program. However, she constantly feels weak and tired. When she walks, she feels pain in her calf muscles. On this visit to her nutritionist, they discuss the vitamin content of her diet and its role in energy metabolism.
Al M. has been hospitalized for congestive heart failure (see Chapter 8) and for head injuries sustained while driving under the influence of alcohol (Chapters 9 and 10). He completed an alcohol detoxification program, enrolled in a local Alcoholics Anonymous (AA) group, and began seeing a psychologist. During this time, his alcohol-related neurological and cardiac manifestations of alcohol toxicity and thiamin deficiency partially cleared. However, in spite of the support he was receiving, he began drinking excessive amounts of alcohol again while eating poorly. Three weeks later, he was readmitted with symptoms of “high-output” heart failure, sometimes referred to as wet beriberi or as the beriberi heart when related to thiamin deficiency.
I. Overview of the Tricarboxylic Acid Cycle
The tricarboxylic acid (TCA) cycle is frequently called the Krebs cycle because Sir Hans Krebs first formulated its reactions into a cycle. It is also called the citric acid cycle because citrate was one of the first compounds known to participate. The most common name for this pathway, the tricarboxylic acid or TCA cycle, denotes the involvement of the tricarboxylates citrate and isocitrate.
For the body to generate large amounts of ATP, the major pathways of fuel oxidation generate acetyl CoA, which is the substrate for the TCA cycle. In the first step of the TCA cycle, the acetyl portion of acetyl CoA combines with the four-carbon intermediate oxaloacetate to form citrate (six carbons), which is rearranged to form isocitrate. In the next two oxidative decarboxylation reactions, electrons are transferred to NAD+ to form NADH, and two molecules of electron-depleted CO2 are released. Subsequently, a high-energy phosphate bond in GTP is generated from substrate-level phosphorylation. In the remaining portion of the TCA cycle, succinate is oxidized to oxaloacetate with the generation of one FAD(2H) and one NADH. The net reaction of the TCA cycle, which is the sum of the equations for individual steps, shows that two carbons of the acetyl group have been entered the cycle, and two molecules of CO2 are produced, with conservation of energy as three molecules of NADH, one of FAD(2H), and one of GTP.
The TCA cycle requires a large number of vitamins and minerals to function. These include niacin (NAD+), riboflavin (FAD and FMN), pantothenic acid (coenzyme A), thiamin, Mg2+, Ca2+, Fe2+, and phosphate.
II. Reactions of the Tricarboxylic Acid Cycle
In the TCA cycle, the two-carbon acetyl group of acetyl CoA enters the cycle, and two oxidized carbons are produced as CO2 (see Fig. 23.1). The function of the cycle is to conserve the energy from this oxidation, which it accomplishes principally by transferring electrons from intermediates of the cycle to NAD+ and FAD. The eight electrons donated by the acetyl group (four from each carbon) eventually end up in three molecules of NADH and one of FAD(2H) (Fig. 23.2). As a consequence, ATP can be generated from oxidative phosphorylation when NADH and FAD(2H) donate these electrons to O2 via the electron transport chain.
FIGURE 23.2 The acetyl group of acetyl CoA. Acetyl CoA donates eight electrons to the TCA cycle, which are shown in red, and two carbons. The high-energy bond is shown by a ~. The acetyl group is the ultimate source of the carbons in the two molecules of CO2 that are produced, and the source of electrons in the one molecule of reduced flavin adenine dinucleotide (FAD[2H]) and three molecules of reduced nicotinamide adenine dinucleotide (NADH), which have each accepted two electrons. However, the same carbon atoms and electrons that enter from one molecule of acetyl CoA do not leave as CO2, NADH, or FAD(2H) within the same turn of the cycle. Acetyl CoA, acetyl coenzyme A; SCoA, coenzyme A; TCA, tricarboxylic acid.
Initially, the acetyl group is incorporated into citrate, an intermediate of the TCA cycle (Fig. 23.3). As citrate progresses through the cycle to oxaloacetate, it is oxidized by four dehydrogenases (isocitrate dehydrogenase, α-ketoglutarate dehydrogenase, succinate dehydrogenase, and malate dehydrogenase), which remove electron-containing hydrogen or hydride atoms from a substrate and transfer them to electron-accepting coenzymes such as NAD+ or FAD. The isomerase aconitase rearranges electrons in citrate, thereby forming isocitrate, to facilitate an electron transfer to NAD+. An iron cofactor in aconitase facilitates the isomerization.
FIGURE 23.3 Reactions of the TCA cycle. The oxidation–reduction enzymes and coenzymes are shown in red. Entry of the two carbons of acetyl CoA into the TCA cycle are indicated with the green box. The carbons released as CO2 are shown with yellow boxes. Acetyl CoA, acetyl coenzyme A; ATP, adenosine triphosphate; CoASH, coenzyme A; FAD, flavin adenine dinucleotide; GDP, guanosine diphosphate; GTP, guanosine triphosphate; NAD, nicotinamide adenine dinucleotide; Pi, inorganic phosphate; TCA, tricarboxylic acid; SCoA, coenzyme A.
Although no oxygen is introduced into the TCA cycle, the two molecules of CO2 produced have more oxygen than the acetyl group. These oxygen atoms are ultimately derived from the carbonyl group of acetyl CoA, two molecules of water added by fumarase and citrate synthase, and the PO42− added to GDP.
The overall yield of energy-containing compounds from the TCA cycle is three NADH, one FAD(2H), and one GTP. The high-energy phosphate bond of GTP is generated from substrate-level phosphorylation catalyzed by succinate thiokinase (succinyl CoA synthetase). As the NADH and FAD(2H) are reoxidized in the electron transport chain, ~2.5 ATP are generated for each NADH, and 1.5 ATP for the FAD(2H) (see Chapter 24). Consequently, the net energy yield from the TCA cycle and oxidative phosphorylation is about 10 high-energy phosphate bonds for each acetyl group oxidized.
A. Formation and Oxidation of Isocitrate
The TCA cycle begins with the condensation of the activated acetyl group and oxaloacetate to form the six-carbon intermediate citrate, a reaction that is catalyzed by the enzyme citrate synthase (see Fig. 23.3). Synthases, in general, catalyze the condensation of two organic molecules to form a carbon–carbon bond in the absence of high-energy phosphate bond energy. A synthetase catalyzes the same type of reaction but requires high-energy phosphate bonds to complete the reaction. Because oxaloacetate is regenerated with each turn of the cycle, it is not really considered a substrate of the cycle, or a source of electrons or carbon.
In the next step of the TCA cycle, the hydroxyl (alcohol) group of citrate is moved to an adjacent carbon so that it can be oxidized to form a keto group. The isomerization of citrate to isocitrate is catalyzed by the enzyme aconitase, which is named for an intermediate of the reaction. The enzyme isocitrate dehydrogenase catalyzes the oxidation of the alcohol group and the subsequent cleavage of the carboxyl group to release CO2 (an oxidation followed by a decarboxylation), forming α-ketoglutarate.
B. α-Ketoglutarate to Succinyl Coenzyme A
The next step of the TCA cycle is the oxidative decarboxylation of α-ketoglutarate to succinyl CoA, catalyzed by the α-ketoglutarate dehydrogenase complex (see Fig. 23.3). The dehydrogenase complex contains the coenzymes thiamin pyrophosphate (TPP), lipoic acid, and FAD.
In this reaction, one of the carboxyl groups of α-ketoglutarate is released as CO2, and the adjacent keto group is oxidized to the level of an acid, which then combines with the sulfhydryl group of coenzyme A (CoASH) to form succinyl CoA (see Fig. 23.3). Energy from the reaction is conserved principally in the reduction state of NADH, with a smaller amount present in the high-energy thioester bond of succinyl CoA.
C. Generation of Guanosine Triphosphate
Energy from the succinyl CoA thioester bond is used to generate GTP from GDP and inorganic phosphate (Pi) in the reaction catalyzed by succinate thiokinase (also known as succinyl CoA synthetase, for the reverse reaction) (see Fig. 23.3). This reaction is an example of substrate-level phosphorylation. By definition, substrate-level phosphorylation is the formation of a high-energy phosphate bond where none previously existed without the use of molecular O2 (in other words, not oxidative phosphorylation). The high-energy phosphate bond of GTP is energetically equivalent to that of ATP and can be used directly for energy-requiring reactions like those involved in protein synthesis.
D. Oxidation of Succinate to Oxaloacetate
Up to this stage of the TCA cycle, two carbons have been stripped of their available electrons and released as CO2. Two pairs of these electrons have been transferred to two NAD+, and one GTP has been generated. However, two additional pairs of electrons arising from acetyl CoA still remain in the TCA cycle as part of succinate. The remaining steps of the TCA cycle transfer these two pairs of electrons to FAD and NAD+ and add H2O, thereby regenerating oxaloacetate.
The sequence of reactions converting succinate to oxaloacetate begins with the oxidation of succinate to fumarate (see Fig. 23.3). Single electrons are transferred from the two adjacent –CH2– methylene groups of succinate to an FAD bound to succinate dehydrogenase, thereby forming the double bond of fumarate. From the reduced enzyme-bound FAD, the electrons are passed into the electron transport chain. A hydroxyl group and a proton from water add to the double bond of fumarate, converting it to malate. In the last reaction of the TCA cycle, the alcohol group of malate is oxidized to a keto group through the donation of electrons to NAD+.
With the regeneration of oxaloacetate, the TCA cycle is complete; the chemical bond energy, carbon, and electrons donated by the acetyl group have been converted to CO2, NADH, FAD(2H), GTP, and heat.
The succinate-to-oxaloacetate sequence of reactions—oxidation through the formation of a double bond, addition of water to the double bond, and oxidation of the resultant alcohol to a ketone—is found in a number of oxidative pathways in the cell, such as the pathways for the oxidation of fatty acids and oxidation of the branched-chain amino acids.
III. Coenzymes of the Tricarboxylic Acid Cycle
The enzymes of the TCA cycle rely heavily on coenzymes for their catalytic function. Isocitrate dehydrogenase and malate dehydrogenase use NAD+ as a coenzyme, and succinate dehydrogenase uses FAD. Citrate synthase catalyzes a reaction that uses a CoA derivative, acetyl CoA. The α-ketoglutarate dehydrogenase complex uses TPP, lipoate, and FAD as bound coenzymes, and NAD+ and CoASH as substrates. Each of these coenzymes has unique structural features that enable it to fulfill its role in the TCA cycle.
A. Flavin Adenine Dinucleotide and NAD+
Both FAD and NAD+ are electron-accepting coenzymes. Why is FAD used in some reactions and NAD+ in others? Their unique structural features enable FAD and NAD+ to act as electron acceptors in different types of reactions and to play different physiological roles in the cell. FAD is able to accept single electrons (H•) and forms a half-reduced single-electron intermediate (Fig. 23.4). It thus participates in reactions in which single electrons are transferred independently from two different atoms, which occurs in double-bond formation (e.g., succinate to fumarate) and disulfide bond formation (e.g., lipoate to lipoate disulfide in the α-ketoglutarate dehydrogenase reaction). In contrast, NAD+ accepts a pair of electrons as the hydride ion (H−), which is attracted to the carbon opposite the positively charged pyridine ring (Fig. 23.5). This occurs, for example, in the oxidation of alcohols to ketones by malate dehydrogenase and isocitrate dehydrogenase. The nicotinamide ring accepts a hydride ion from the C–H bond, and the alcoholic hydrogen is released into the medium as a positively charged proton, H+.
FIGURE 23.4 One-electron steps in the reduction of FAD. When FAD and FMN accept single electrons, they are converted to the half-reduced semiquinone, a semistable free-radical form. They can also accept two electrons to form the fully reduced form, FADH2. However, in most dehydrogenases, FADH2 is never formed. Instead, the first electron is shared with a group on the protein as the next electron is transferred. Therefore, in this text, overall acceptance of two electrons by FAD has been denoted by the more general abbreviation, FAD(2H). FAD, flavin adenine dinucleotide; FMN, flavin mononucleotide.
FIGURE 23.5 Oxidation and decarboxylation of isocitrate. The alcohol group (C–OH) is oxidized to a ketone, with the C–H electrons donated to NAD+ as the hydride ion. Subsequent electron shifts in the pyridine ring remove the positive charge. The H of the –OH group dissociates into water as a proton, H+. NAD+, the electron acceptor, is reduced. NAD+, nicotinamide adenine dinucleotide.
The free-radical, single-electron forms of FAD are very reactive, and FADH can lose its electron through exposure to water or the initiation of chain reactions. As a consequence, FAD must remain very tightly, sometimes covalently, attached to its enzyme while it accepts and transfers electrons to another group bound on the enzyme. Because FAD interacts with many functional groups on amino acid side chains in the active site, the E0′ for enzyme-bound FAD varies greatly and can be greater or much less than that of NAD+. In contrast, NAD+ and NADH are more like substrate and product than coenzymes.
NADH plays a regulatory role in balancing energy metabolism that FAD(2H) cannot because FAD(2H) remains attached to its enzyme. Free NAD+ binds to a dehydrogenase and is reduced to NADH, which is then released into the medium, where it can bind and inhibit a different dehydrogenase. Consequently, oxidative enzymes are controlled by the NADH/NAD+ ratio and do not generate NADH faster than it can be reoxidized in the electron transport chain. The regulation of the TCA cycle and other pathways of fuel oxidation by the NADH/NAD+ ratio is part of the mechanism for coordinating the rate of fuel oxidation to the rate of ATP utilization.
B. Role of Coenzyme A in the Tricarboxylic Acid Cycle
Coenzyme A (CoASH), the acylation coenzyme, participates in reactions through the formation of a thioester bond between the sulfur (S) of CoASH and an acyl group (e.g., acetyl CoA, succinyl CoA) (Fig. 23.6). The complete structure of CoASH and its vitamin precursor, pantothenate, is shown in Figure 8.9A. A thioester bond differs from a typical oxygen ester bond because S, unlike O, does not share its electrons and participate in resonance formations. One of the consequences of this feature of sulfur chemistry is that the carbonyl carbon, the α-carbon, and the β-carbon of the acyl group in a CoA thioester can be activated for participation in different types of reactions (e.g., in the citrate synthase reaction, the α-carbon methyl group is activated for condensation with oxaloacetate; see Figs. 23.3 and 23.6A). Another consequence is that the thioester bond is a high-energy bond that has a large negative ΔG0′ of hydrolysis (~−13 kcal/mol).
FIGURE 23.6 Utilization of the high-energy thioester bond of acyl CoAs. Energy transformations are shown in red. A. The energy released by hydrolysis of the thioester bond of acetyl CoA in the citrate synthase reaction contributes a large negative ΔG0′ to the forward direction of the TCA cycle. B. The energy of the succinyl CoA thioester bond is used for the synthesis of the high-energy phosphate bond of GTP. Acetyl CoA, acetyl coenzyme A; GTP, guanosine triphosphate; SCoA, coenzyme A; TCA, tricarboxylic acid.
The energy from cleavage of the high-energy thioester bonds of succinyl CoA and acetyl CoA is used in two different ways in the TCA cycle. When the succinyl CoA thioester bond is cleaved by succinate thiokinase, the energy is used directly for activating an enzyme-bound phosphate that is transferred to GDP (see Fig. 23.6B). In contrast, when the thioester bond of acetyl CoA is cleaved in the citrate synthase reaction, the energy is released, giving the reaction a large negative ΔG0′ of −7.7 kcal/mol. The large negative ΔG0′ for citrate formation helps to keep the TCA cycle going in the forward direction.
C. The α-Keto Acid Dehydrogenase Complexes
The α-ketoglutarate dehydrogenase complex is one of a three-member family of similar α-keto acid dehydrogenase complexes. The other members of this family are the pyruvate dehydrogenase complex and the branched-chain amino acid α-keto acid dehydrogenase complex. Each of these complexes is specific for a different α-keto acid structure. In the sequence of reactions catalyzed by the complexes, the α-keto acid is decarboxylated (i.e., releases the carboxyl group as CO2) (Fig. 23.7). The keto group is oxidized to the level of a carboxylic acid and then combined with CoASH to form an acyl CoA thioester (e.g., succinyl CoA).
FIGURE 23.7 Oxidative decarboxylation of α-ketoglutarate. The α-ketoglutarate dehydrogenase complex oxidizes α-ketoglutarate to succinyl coenzyme A (succinyl CoA). The carboxyl group is released as CO2. The keto group on the α-carbon is oxidized and then forms the acyl CoA thioester, succinyl CoA. The α, β, γ, and δ on succinyl CoA refer to the sequence of atoms in α-ketoglutarate. CoASH, coenzyme A; FAD, flavin adenine dinucleotide; NAD, nicotinamide adenine dinucleotide; SCoA, coenzyme A.
All of the α-keto acid dehydrogenase complexes are huge enzyme complexes composed of multiple subunits of three different enzymes, E1, E2, and E3. E1 is an α-keto acid decarboxylase, which contains thiamin pyrophosphate (TPP); it cleaves off the carboxyl group of the α-keto acid. E2 is a transacylase containing lipoate; it transfers the acyl portion of the α-keto acid from thiamin to CoASH. E3 is dihydrolipoyl dehydrogenase, which contains FAD; it transfers electrons from reduced lipoate to FAD, which then transfers the electrons to NAD+. The collection of three enzyme activities into one huge complex enables the product of one enzyme to be transferred to the next enzyme without loss of energy. Complex formation also increases the rate of catalysis because the substrates for E2 and E3 remain bound to the enzyme complex. The mechanism of an oxidative decarboxylation is depicted in Figures e-23.1 and e-23.2 .
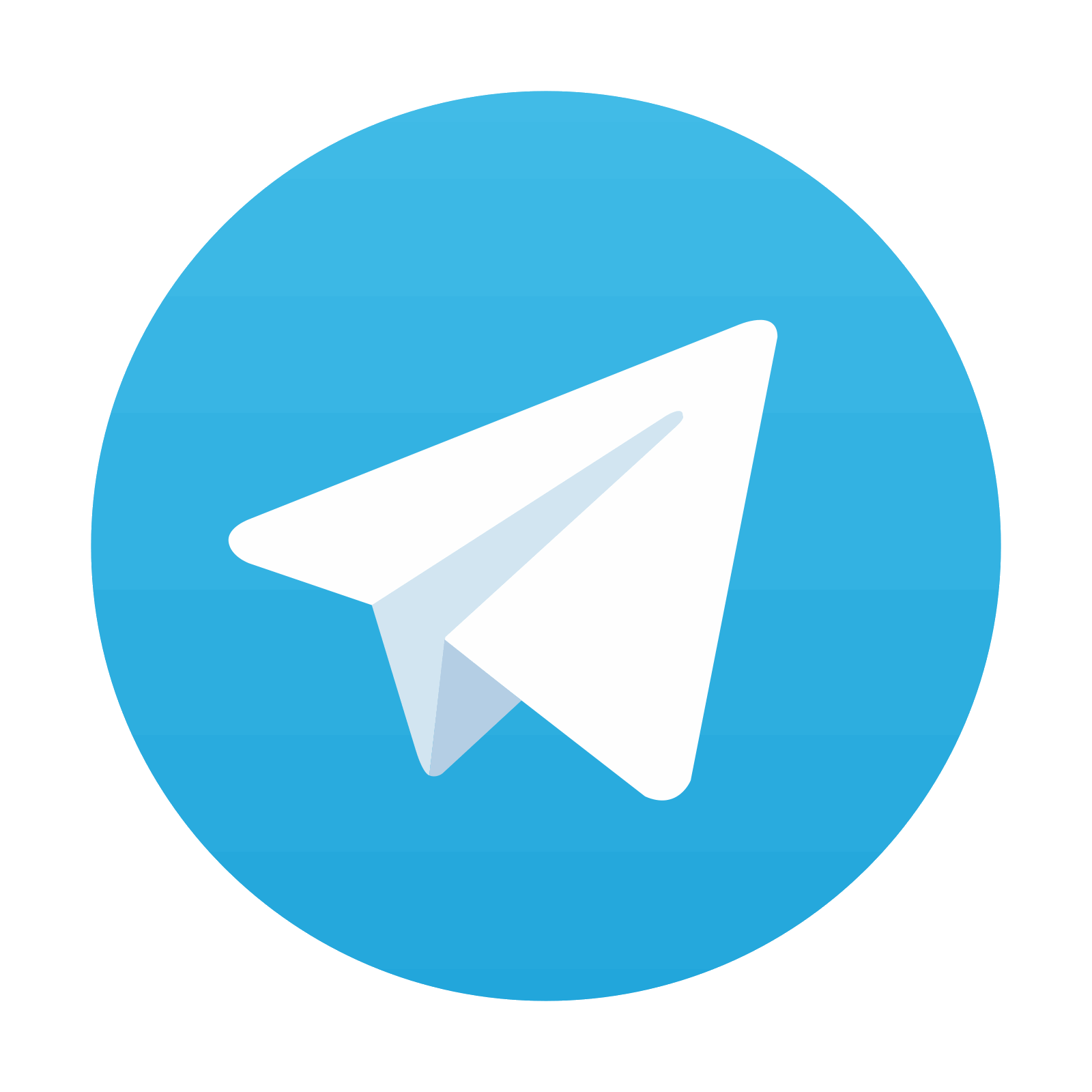
Stay updated, free articles. Join our Telegram channel
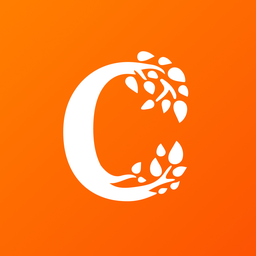
Full access? Get Clinical Tree
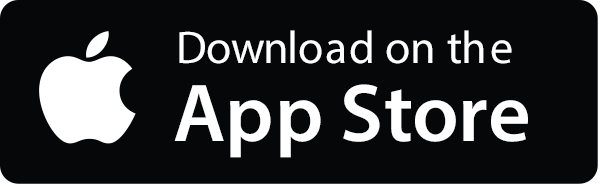
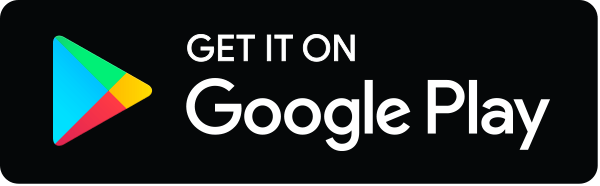