The nervous system consists of various cell types. The most abundant cell in the nervous system is the glial cell, which consists of astrocytes and oligodendrocytes in the central nervous system, and Schwann cells in the peripheral nervous system. These cells provide support for the neurons and synthesize the protective myelin sheath that surrounds the axons emanating from the neurons. Microglial cells in the nervous system act as immune cells, destroying and ingesting foreign organisms that enter the nervous system. The interface between the brain parenchyma and the cerebrospinal fluid compartment is formed by the ependymal cells, which line the cavities of the brain and spinal cord. These cells use their cilia to allow for the circulation of the cerebrospinal fluid (CSF), which bathes the cells of the central nervous system.
The cells of the brain are separated from free contact with the rest of the body by the blood–brain barrier. The capillaries of the brain exhibit features, such as tight endothelial cell junctions, that restrict their permeability to metabolites in the blood. This protects the brain from compounds that might be toxic or otherwise interfere with nerve impulse transmission. It also affects the entry of precursors for brain metabolic pathways such as fuel metabolism and neurotransmitter synthesis.
Neurotransmitters can be divided structurally into two categories: small nitrogen-containing neurotransmitters and neuropeptides. The small nitrogen-containing neurotransmitters are generally synthesized in the presynaptic terminal from amino acids and intermediates of glycolysis and the tricarboxylic acid (TCA) cycle. They are retained in storage vesicles until the neuron is depolarized. The catecholamine neurotransmitters (dopamine, norepinephrine, and epinephrine) are derived from tyrosine. Serotonin is synthesized from tryptophan. Acetylcholine is synthesized from choline, which can be supplied from the diet or is synthesized and stored as part of phosphatidylcholine. Glutamate and its neurotransmitter derivative, γ-aminobutyric acid (GABA), are derived from α–ketoglutarate in the TCA cycle. Glycine is synthesized in the brain from serine. The synthesis of the neurotransmitters is regulated to correspond to the rate of depolarization of the individual neurons. A large number of cofactors are required for the synthesis of neurotransmitters, and deficiencies of pyridoxal phosphate, thiamin pyrophosphate, and vitamin B12 result in a variety of neurological dysfunctions.
Brain metabolism has a high requirement for glucose and oxygen. Deficiencies of either (hypoglycemia or hypoxia) affect brain function because they influence adenosine triphosphate (ATP) production and the supply of precursors for neurotransmitter synthesis. Ischemia elicits a condition in which increased calcium levels, swelling, glutamate excitotoxicity, and nitric oxide generation affect brain function, and can lead to a stroke. The generation of free radicals and abnormalities in nitric oxide production are important players in the pathogenesis of a variety of neurodegenerative diseases.
Because of the restrictions posed by the blood–brain barrier to the entry of a variety of substances into the central nervous system, the brain generally synthesizes and degrades its own lipids. Essential fatty acids can enter the brain, but the more common fatty acids do not. The turnover of lipids at the synaptic membrane is very rapid, and the neuron must replace those lipids lost during exocytosis. The glial cells produce the myelin sheath, which is composed primarily of lipids. These lipids are of a different composition than those of the neuronal cells. Because there is considerable lipid synthesis and turnover in the brain, this organ is sensitive to disorders of peroxisomal function (Refsum disease; interference in very-long-chain fatty acid oxidation and α-oxidation) and lysosomal diseases (mucopolysaccharidoses; inability to degrade complex lipids and glycolipids).
THE WAITING ROOM 
Katie C., a 34-year-old dress designer, developed alarming palpitations of her heart while bending forward to pick up her cat. She also developed a pounding headache and started sweating profusely. After 5 to 10 minutes, these symptoms subsided. One week later, her aerobic exercise instructor, a registered nurse, noted that Katie C. grew very pale and was tremulous during exercise. The instructor took Katie C.’s blood pressure, which was 220 mm Hg systolic (normal, up to 120 at rest) and 132 mm Hg diastolic (normal, up to 80 at rest). Within 15 minutes, Katie C. recovered, and her blood pressure returned to normal. The instructor told Katie C. to see her physician the next day.
The doctor told Katie C. that her symptom complex coupled with severe hypertension was concerning for a pheochromocytoma, a tumor in the medulla (central area) of the adrenal glands, which can episodically secrete large amounts of catecholamines, such as norepinephrine (noradrenaline) and epinephrine (adrenaline). Her blood pressure was normal until moderate pressure to the left of her umbilicus caused her to suddenly develop a typical attack, and her blood pressure rose rapidly. In addition to ordering several biochemical tests, she also was scheduled for a magnetic resonance imaging (MRI) study of her abdomen and pelvis. The MRI showed a 3.5 × 2.8 × 2.6 cm mass in the left adrenal gland, with imaging characteristics typical of a pheochromocytoma.
Ivan A.’s brother, Evan A., was 6 ft tall and weighed 425 lb (BMI = 57.6 kg/m2). He had only been successful in losing weight once in his life, in 1977, when he had lost 30 lb through a combination of diet and exercise with the support of a registered dietician. However, he quickly regained this weight over the next year. Evan A.’s weight was not usually a concern for him, but in 1997 he became worried when it became difficult for him to take walks or go fishing because of joint pain in his knees. He was also suffering from symptoms suggestive of a peripheral neuropathy, manifesting primarily as tingling in his legs. In 1997, Evan A. approached his physician desperate for help to lose weight. The physician placed Evan A. on a new drug, Redux, which had just been approved for use as a weight-loss agent, and a low-fat, low-calorie diet along with physical therapy to help increase his activity level. In 4 months, Evan A.’s weight dropped from 425 to 375 lb, his total cholesterol dropped from 250 to 185, and his serum triglycerides dropped from 375 to 130. However, Redux was withdrawn from the market by its manufacturer late in 1997 because of reports that it caused cardiovascular adverse events and abnormalities in the structure of heart valve tissue. Evan A. was then placed on Prozac, a drug used primarily as an antidepressant, which may cause mild weight loss.
I. Cell Types of the Nervous System
The nervous system consists of neurons, the cells that transmit signals, and supporting cells, the neuroglia. The neuroglia consists of oligodendrocytes and astrocytes (known collectively as glial cells), microglial cells, ependymal cells, and Schwann cells. The neuroglia are designed to support and sustain the neurons and do so by surrounding neurons and holding them in place, supplying nutrients and oxygen to the neurons, insulating neurons so their electrical signals are more rapidly propagated, and cleaning up any debris that enters the nervous system. The central nervous system (CNS) consists of the brain and spinal cord. This system integrates all signals emanating from the peripheral nervous system (PNS). The PNS is composed of all neurons that lie outside the CNS.
A. Neurons
Neurons consist of a cell body (soma) from which long (axons) and short (dendrites) extensions protrude. Dendrites receive information from the axons of other neurons, whereas the axons transmit information to other neurons. The axon–dendrite connection is known as a synapse (Fig. 46.1). Most neurons contain multiple dendrites, each of which can receive signals from multiple axons. This configuration allows a single neuron to integrate information from multiple sources. Although neurons also contain just one axon, most axons branch extensively and distribute information to multiple targets (divergence). The neurons transmit signals by changes in the electrical potential across their membrane. Signaling across a synapse requires the release of neurotransmitters that, when bound to their specific receptors, initiate an electrical signal in the receiving or target cell. Neurons are terminally differentiated cells and, as such, have little capability for division. As a result, injured neurons have a limited capacity to repair themselves and frequently undergo apoptosis (programmed cell death) when damaged.
FIGURE 46.1 A neuron consists of a cell body (soma) with short extensions (dendrites) and a long extension (axon). The axon–dendrite interface is the synapse. A soma may receive input from multiple axons.
B. Neuroglial Cells
1. Astrocytes
The astrocytes are found in the CNS and are star-shaped cells that provide physical and nutritional support for neurons. During the development of the CNS, the astrocytes guide neuronal migration to their final adult position and form a matrix that keeps neurons in place. These cells serve several functions, including phagocytosing debris left behind by cells, providing lactate (from glucose metabolism) as a carbon source for the neurons, and controlling the brain extracellular ionic environment. Astrocytes help to regulate the content of the extracellular fluid (ECF) by taking up, processing, and metabolizing nutrients and waste products.
2. Oligodendrocytes
Oligodendrocytes provide the myelin sheath that surrounds the axon, acting as “insulation” for many of the neurons in the CNS. The myelin sheath is the lipid–protein covering of the axons (see Section V.B for a description of the composition and synthesis of the myelin sheath). Oligodendrocytes can form myelin sheaths around multiple neurons in the CNS by sending out processes that bind to the axons on target neurons. The speed with which a neuron conducts its electric signal (action potential) is directly proportional to the degree of myelination. Oligodendrocytes, along with the astrocytes, form a supporting matrix for the neurons. Oligodendrocytes have a limited capacity for mitosis, and if damaged, do not replicate. If this occurs, demyelination of the axons may occur, resulting in abnormalities in signal conduction along that axon (see “Biochemical Comments”).
3. Schwann Cells
Schwann cells are the supporting cells of the PNS. Like oligodendrocytes, Schwann cells form myelin sheaths around the axons, but unlike the oligodendrocytes, Schwann cells myelinate only one axon. Schwann cells also clean up cellular debris in the PNS. The Schwann cells also provide a means for peripheral axons to regenerate if damaged. There is a synergistic interaction among the Schwann cells, secreted growth factors, and the axon that allows damaged axons to reconnect to the appropriate target axon.
4. Microglial Cells
The microglial cells are the smallest glial cells in the nervous system. They serve as immunologically responsive cells that function similarly to the action of macrophages in the circulation. Microglial cells destroy invading microorganisms and phagocytose cellular debris.
5. Ependymal Cells
The ependymal cells are ciliated cells that line the cavities (ventricles) of the CNS and the spinal cord. In some areas of the brain, the ependymal cells are functionally specialized to elaborate and secrete cerebrospinal fluid (CSF) into the ventricular system. The beating of the ependymal cilia allow for efficient circulation of the CSF throughout the CNS. The CSF acts as both a shock absorber protecting the CNS from mechanical trauma and as a system for the removal of metabolic wastes. The CSF can be aspirated from the spinal canal and analyzed to determine whether disorders of CNS function, with their characteristic CSF changes, are present.
For many years, it had been believed that damaged neurons in the CNS could not regenerate because it was thought that there are no pluripotent stem cells (cells that can differentiate into various cell types found in the CNS) in the CNS. However, recent data suggest that cells found within the ependymal layer can act as neural stem cells, which under appropriate stimulation can regenerate cells within the nervous system. Such a finding has led to significant research looking for potential treatments for diseases that alter neuronal cell function.
II. THE BLOOD–BRAIN BARRIER
A. Capillary Structure
In the capillary beds of most organs, rapid passage of molecules occurs from the blood through the endothelial wall of the capillaries into the interstitial fluid. Thus, the composition of interstitial fluid resembles that of blood, and specific receptors or transporters in the plasma membrane of the cells being bathed by the interstitial fluid may interact directly with amino acids, hormones, or other compounds from the blood. In the brain, transcapillary movement of substrates in the peripheral circulation into the brain is highly restricted by the blood–brain barrier. This barrier limits the accessibility of blood-borne toxins and other potentially harmful compounds to the neurons of the CNS.
The blood–brain barrier begins with the endothelial cells that form the inner lining of the vessels supplying blood to the CNS (Fig. 46.2). Unlike the endothelial cells of other organs, these cells are joined by tight junctions that do not permit the movement of polar molecules from the blood into the interstitial fluid bathing the neurons. They also lack mechanisms for transendothelial transport that are present in other capillaries of the body. These mechanisms include fenestrations (“windows” or pores that span the endothelial lining and permit the rapid movement of molecules across membranes) or transpinocytosis (vesicular transport from one side of the endothelial cell to another).
FIGURE 46.2 The blood–brain barrier. Compounds in the blood cannot pass freely into the brain; they must traverse the endothelial cells, basement membrane, and astrocytes, using specific carriers to gain access to the brain. Very lipophilic molecules may pass through all of these membranes in the absence of a carrier.
The endothelial cells serve actively, as well as passively, to protect the brain. Because they contain a variety of drug-metabolizing enzyme systems similar to the drug-metabolizing enzymes found in the liver, the endothelial cells can metabolize neurotransmitters and toxic chemicals and, therefore, form an enzymatic barrier to entry of these potentially harmful substances into the brain. They actively pump hydrophobic molecules that diffuse into endothelial cells back into the blood (especially xenobiotics) with P-glycoproteins, which act as transmembranous, ATP-dependent efflux pumps. Although lipophilic substances, water, oxygen, and carbon dioxide can readily cross the blood–brain barrier by passive diffusion, other molecules depend on specific transport systems. Differential transporters on the luminal and abluminal endothelial membranes can transport compounds into, as well as out of, the brain.
Further protection against the free entry of blood-borne compounds into the CNS is provided by a continuous collagen-containing basement membrane that completely surrounds the capillaries. The basement membrane appears to be surrounded by the foot processes of astrocytes. Thus, compounds must pass through endothelial cell membranes, the enzymatic barrier in the endothelial cells, the basement membrane, and possibly additional cellular barriers formed by the astrocytes to reach the neurons in the brain.
B. Transport through the Blood–Brain Barrier
Many nonpolar substances, such as drugs and inert gases, probably diffuse through the endothelial cell membranes. A large number of other compounds are transported through the endothelial capillaries by facilitative transport, whereas others, such as nonessential fatty acids, cannot cross the blood–brain barrier. Essential fatty acids, however, are transported across the barrier.
1. Fuels
Glucose, which is the principal fuel of the brain, is transported through both endothelial membranes by facilitated diffusion via the GLUT-1 transporter (see Fig. 21.9 and Table 21.5). GLUT-3 transporters present on the neurons then allow the neurons to transport the glucose from the ECF. Glial cells express GLUT-1 transporters. Although the rate of glucose transport into the ECF normally exceeds the rate required for energy metabolism by the brain, glucose transport may become rate-limiting as blood glucose levels fall below the normal range. Thus, individuals begin to experience hypoglycemic symptoms at glucose levels of approximately 60 mg/dL, as the glucose levels are reduced to the Km, or below the Km values of the GLUT-1 transporters in the endothelial cells of the barrier.
Monocarboxylic acids, including L-lactate, acetate, pyruvate, and the ketone bodies acetoacetate and β-hydroxybutyrate, are transported by a separate stereospecific system that is slower than the transport system for glucose. During starvation, when the level of ketone bodies in the blood is elevated, this transporter is upregulated. Ketone bodies are important fuels for the brain of both adults and neonates during prolonged starvation (>46 hours).
2. Amino Acids and Vitamins
Large neutral amino acids (LNAAs, such as phenylalanine, leucine, tyrosine, isoleucine, valine, tryptophan, methionine, and histidine) enter the CSF rapidly via a single amino acid transporter (L-[leucine preferring]-system amino acid transporter). Many of these compounds are essential in the diet and must be imported for protein synthesis or as precursors of neurotransmitters. Because a single transporter is involved, these amino acids compete with each other for transport into the brain.
The entry of small neutral amino acids, such as alanine, glycine, proline, and GABA, is markedly restricted because their influx could dramatically change the content of neurotransmitters (see Section III). They are synthesized in the brain, and some are transported out of the CNS and into the blood via the A-(alanine-preferring)-system carrier. Vitamins have specific transporters through the blood–brain barrier, just as they do in most tissues.
3. Receptor-Mediated Transcytosis
Certain proteins, such as insulin, transferrin, and insulin-like growth factors, cross the blood–brain barrier by receptor-mediated transcytosis. Once the protein binds to its membrane receptor, the membrane containing the receptor–protein complex is endocytosed into the endothelial cell to form a vesicle. It is released on the other side of the endothelial cell. Absorptive-mediated transcytosis also can occur. This differs from receptor-mediated transcytosis in that the protein binds nonspecifically to the membrane and not to a distinct receptor.
III. SYNTHESIS OF SMALL NITROGEN-CONTAINING NEUROTRANSMITTERS
Molecules that serve as neurotransmitters fall into two basic structural categories: (1) small nitrogen-containing molecules and (2) neuropeptides. The major small nitrogen-containing molecule neurotransmitters include glutamate, GABA, glycine, acetylcholine, dopamine, norepinephrine, serotonin, and histamine. Additional neurotransmitters that fall into this category include epinephrine, aspartate, and nitric oxide. In general, each neuron synthesizes only those neurotransmitters that it uses for transmission through a synapse or to another cell. The neuronal tracts are often identified by their neurotransmitter; for example, a dopaminergic tract synthesizes and releases the neurotransmitter dopamine.
Neuropeptides are usually small peptides that are synthesized and processed in the CNS. Some of these peptides have targets within the CNS (such as endorphins, which bind to opioid receptors and block pain signals), whereas others are released into the circulation to bind to receptors on other organs (such as growth hormone and thyroid-stimulating hormone). Many neuropeptides are synthesized as a larger precursor, which is then cleaved to produce the active peptides. Until recently, the assumption was that a neuron synthesized and released only a single neurotransmitter. More recent evidence suggests that a neuron may contain (1) more than one small-molecule neurotransmitter, (2) more than one neuropeptide neurotransmitter, or (3) both types of neurotransmitters. The differential release of the various neurotransmitters is the result of the neuron altering its frequency and pattern of firing.
A. General Features of Neurotransmitter Synthesis
Several features are common to the synthesis, secretion, and metabolism of most small nitrogen-containing neurotransmitters (Table 46.1). Most of these neurotransmitters are synthesized from amino acids, intermediates of glycolysis and the TCA cycle, and O2 in the cytoplasm of the presynaptic terminal. The rate of synthesis is generally regulated to correspond to the rate of firing of the neuron. Once they are synthesized, the neurotransmitters are transported into storage vesicles by an ATP-requiring pump linked with the proton gradient. Release from the storage vesicle is triggered by the nerve impulse that depolarizes the postsynaptic membrane and causes an influx of Ca2+ ions through voltage-gated calcium channels. The influx of Ca2+ promotes fusion of the vesicle with the synaptic membrane and release of the neurotransmitter into the synaptic cleft. The transmission across the synapse is completed by binding of the neurotransmitter to a receptor on the postsynaptic membrane (Fig. 46.3).
TABLE 46.1 Features Common to Neurotransmittersa
◆ Synthesis from amino acid and common metabolic precursors usually occurs in the cytoplasm of the presynaptic nerve terminal. The synthetic enzymes are transported by fast axonal transport from the cell body, where they are synthesized, to the presynaptic terminal. |
◆ The synthesis of the neurotransmitter is regulated to correspond to the rate of firing of the neuron, both acutely and through long-term enhancement of synaptic transmission. |
◆ The neurotransmitter is actively taken up into storage vesicles in the presynaptic terminal. |
◆ The neurotransmitter acts at a receptor on the postsynaptic membrane. |
◆ The action of the neurotransmitter is terminated through reuptake into the presynaptic terminal, diffusion away from the synapse, or enzymatic inactivation. The enzymatic inactivation may occur in the postsynaptic terminal, the presynaptic terminal, or an adjacent astrocyte or microglial cell. |
◆ The blood–brain barrier affects the supply of precursors for neurotransmitter synthesis. |
aNot all neurotransmitters exhibit all of these features. Nitric oxide is an exception to most of these generalities. Some neurotransmitters (epinephrine, serotonin, and histamine) are also secreted by cells other than neurons. Their synthesis and secretion by non-neuronal cells follow other principles.
FIGURE 46.3 Action of neurotransmitters.
Not all neurotransmitters exhibit all of these features. Nitric oxide, because it is a gas, is an exception to most of these generalities. Some neurotransmitters are synthesized and secreted by both neurons and other cells (e.g., epinephrine, serotonin, histamine).
B. Dopamine, Norepinephrine, and Epinephrine
1. Synthesis of the Catecholamine Neurotransmitters
The three neurotransmitters dopamine, norepinephrine, and epinephrine are synthesized in a common pathway from the amino acid L-tyrosine. Tyrosine is supplied in the diet or is synthesized in the liver from the essential amino acid phenylalanine by phenylalanine hydroxylase (see Chapter 37). The pathway of catecholamine biosynthesis is shown in Figure 46.4.
FIGURE 46.4 The pathways of catecholamine and melanin biosynthesis. The dark boxes indicate the enzymes, which, when defective, lead to albinism. BH2, dihydrobiopterin; BH4, tetrahydrobiopterin; Dopa, dihydroxyphenylalanine; PLP, pyridoxal phosphate.
The second step in catecholamine synthesis is the decarboxylation of L-DOPA to form dopamine. This reaction, like many decarboxylation reactions of amino acids, requires pyridoxal phosphate. Dopaminergic neurons (neurons that use dopamine as a neurotransmitter) stop the synthesis at this point because these neurons do not synthesize the enzymes required for the subsequent steps.
Neurons that secrete norepinephrine synthesize it from dopamine in a hydroxylation reaction catalyzed by dopamine β-hydroxylase (DBH). This enzyme is present only within the storage vesicles of these cells. Like tyrosine hydroxylase, it is a mixed-function oxidase that requires an electron donor. Ascorbic acid (vitamin C) serves as the electron donor and is oxidized in the reaction. Copper (Cu2+) is a bound cofactor that is required for the electron transfer.
Although the adrenal medulla is the major site of epinephrine synthesis, epinephrine is also synthesized in a few neurons that use it as a neurotransmitter. These neurons contain the above pathway for norepinephrine synthesis and in addition contain the enzyme that transfers a methyl group from S-adenosyl methionine (SAM) to norepinephrine to form epinephrine. Thus, epinephrine synthesis is dependent on the presence of adequate levels of vitamin B12 and folate to produce the SAM (see Chapter 38).
2. Storage and Release of Catecholamines
Ordinarily, only low concentrations of catecholamines are free in the cytosol, whereas high concentrations are found within the storage vesicles. Conversion of tyrosine to L-DOPA and that of L-DOPA to dopamine occurs in the cytosol. Dopamine is then taken up into the storage vesicles. In norepinephrine-containing neurons, the final β-hydroxylation reaction occurs in the vesicles.
The catecholamines are transported into vesicles by the protein VMAT2 (vesicle monoamine transporter 2) (Fig. 46.5). The vesicle transporters contain 12 transmembrane domains and are homologous to a family of bacterial drug-resistance transporters, including P-glycoprotein. The mechanism that concentrates the catecholamines in the storage vesicles is an ATP-dependent process linked to a proton pump (secondary active transport). Protons are pumped into the vesicles by a vesicular ATPase (V-ATPase). The protons then exchange for the positively charged catecholamine via the transporter VMAT2. The influx of the catecholamine is thus driven by the H+ gradient across the vesicular membrane. The intravesicular concentration of catecholamines is approximately 0.5 M, roughly 100 times the cytosolic concentration. In the vesicles, the catecholamines exist in a complex with ATP and acidic proteins known as chromogranins.
FIGURE 46.5 Transport of catecholamines into storage vesicles. This is a secondary active transport based on the generation of a proton gradient across the vesicular membrane. ADP, adenosine diphosphate; ATP, adenosine triphosphate; DBH, dopamine β-hydroxylase; NT+, positively charged neurotransmitter (catecholamine); V-ATPase, vesicular ATPase; VMAT2, vesicle monoamine transporter 2.
The vesicles play a dual role: They maintain a ready supply of catecholamines at the nerve terminal that is available for immediate release, and they mediate the process of release. When an action potential reaches the nerve terminal, Ca2+ channels open, allowing an influx of Ca2+, which promotes the fusion of vesicles with the neuronal membrane. The vesicles then discharge their soluble contents, including the neurotransmitters, ATP, chromogranins, and DBH, into the extraneuronal space by the process of exocytosis. In some cases, the catecholamines affect other neurons. In other cases, they circulate in the blood and initiate responses in peripheral tissues.
3. Inactivation and Degradation of Catecholamine Neurotransmitters
The action of catecholamines is terminated through reuptake into the presynaptic terminal and diffusion away from the synapse. Degradative enzymes are present in the presynaptic terminal, and in adjacent cells, including glial cells and endothelial cells.
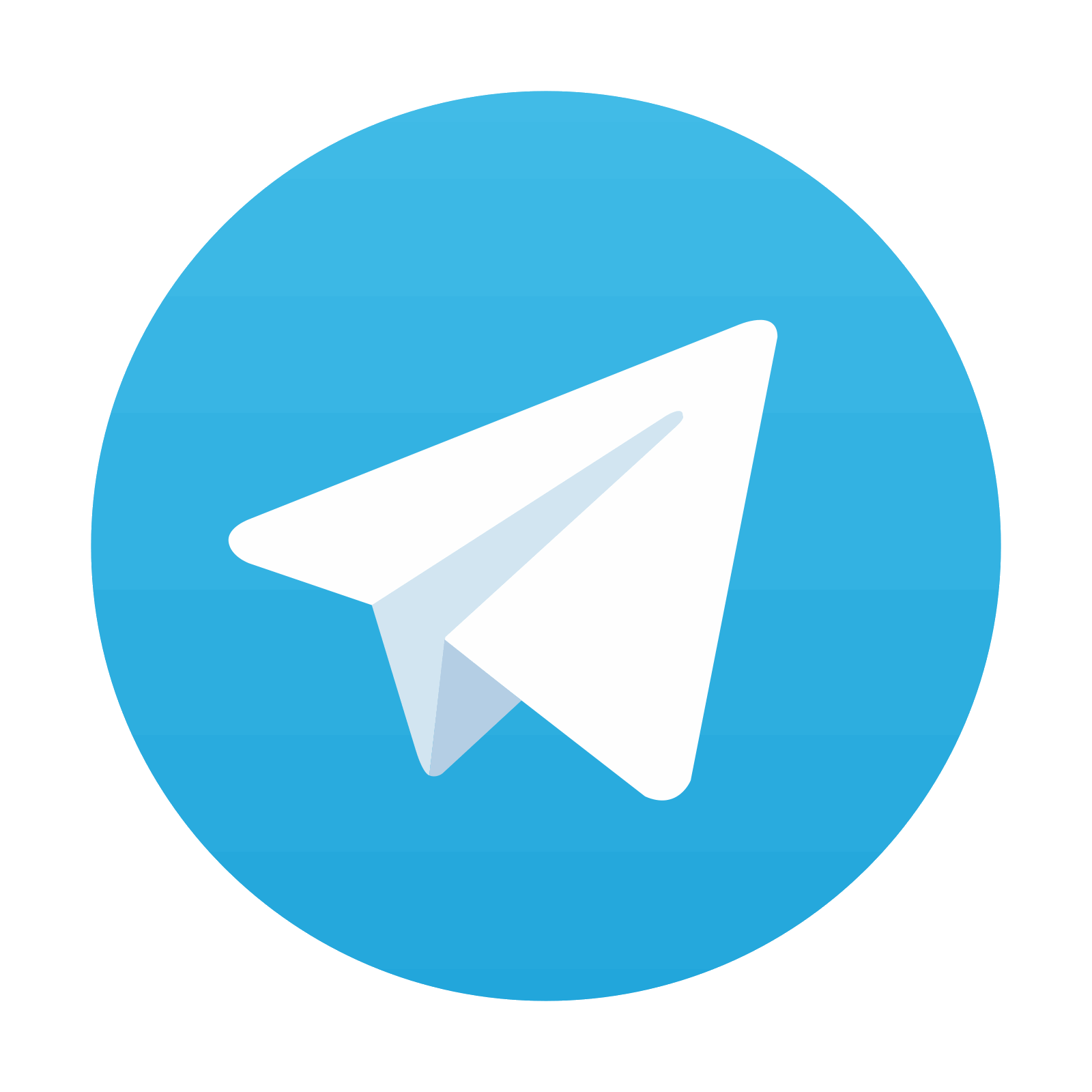
Stay updated, free articles. Join our Telegram channel
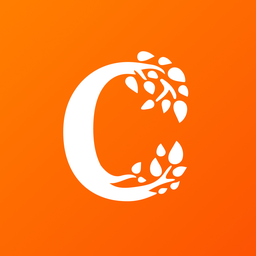
Full access? Get Clinical Tree
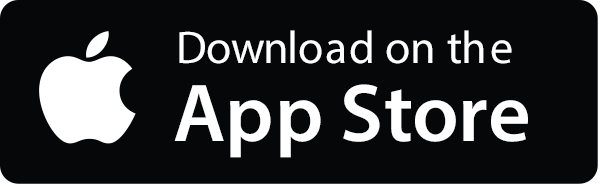
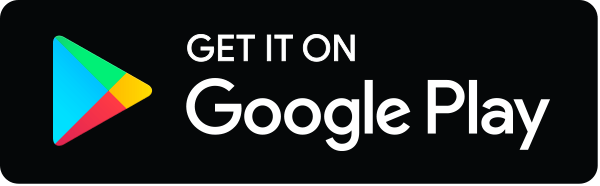