(1)
Faculty of Life Sciences, Bar-Ilan University, Ramat-Gan, Israel
Keywords
Tissue oxygen balanceEnergy demandEnergy supplyATP consumptionOxygen gradient2.1 Tissue Energy Metabolism
The functional capacity of any tissue is related to its ability to perform work. It is possible to assess this ability through the knowledge of changes in the oxygen balance, that is, the ratio of oxygen supply to oxygen demand in the tissue. As seen in Fig. 2.1, this concept was suggested by Barcroft 100 years ago [1].


Fig. 2.1
The hypothesis formulated by Barcroft in 1914 regarding the connection between organ activity, oxygen consumption, and blood flow [1]
Barcroft described the relationship between tissue activity, oxygen consumption, and increase in blood supply as a compensation mechanism. This observation, which was published in 1914, was and is supported widely by many studies published in the past 100 years. Schematic presentation of the balance between oxygen supply and demand in a typical tissue is shown in Fig. 2.2. The supply of oxygen is dependent upon microcirculatory blood flow (TBF), blood volume (TBV), and the level of oxygen bound to the hemoglobin (HbO2) in the small blood vessels, namely, in the microcirculation.


Fig. 2.2
Schematic presentation of tissue energy balance evaluated by energy supply and demand. Energy supply could be measured by monitoring tissue blood flow (TBF), tissue blood volume (TBV), and hemoglobin oxygenation (HbO 2 ), which is similar in all tissues. Energy demand varies between the different tissues and may include ionic homeostasis, signal conduction, muscle contraction, glandular secretion, and GI tract and kidney function. Mitochondrial NADH redox state serves as an indicator for tissue energy or oxygen balance. (© Reprinted with kind permission of Springer Science Business Media [83])
The level of oxygenated hemoglobin in the microcirculation is affected by two factors, namely, oxygen consumption by the mitochondria and microcirculatory blood flow. The demand for oxygen is affected by the specific activities taking place in each organ. The intracellular level of mitochondrial NADH (the reduced form) is a parameter related to oxygen balance.
Figure 2.3 shows the gradient of oxygen in room air through the lungs, the large arteries, and small arterioles to the intracellular space, and finally to the mitochondrion. In this scheme, the various points of patient monitoring are presented. The last usual parameter in the oxygen gradient is the pulse oximeter, which measures the saturation of hemoglobin in the large arteries. As of today, monitoring of microcirculation, and especially mitochondrial function, is not a standard approach and clinical tool. This issue is described in Chap. 9.


Fig. 2.3
Oxygen gradient from air to the mitochondria. Clinical monitoring of patients includes the various parameters along the oxygen gradient. (© Reprinted with kind permission of Springer Science Business Media [84])
Figure 2.4d presents the involvement of the mitochondria in cellular and tissue energy metabolism. Substrates and O2 are supplied and are regulated by the blood in the microcirculation, namely, from very small arterioles and the capillary bed. The main function of the mitochondria is to convert the potential energy stored in various substrates and its metabolites (e.g., glucose) into ATP. The inner membrane of the mitochondria contains five complexes of integral membrane proteins, including NADH dehydrogenase (Complex 1). Three of those proteins are involved in the respiratory chain activity. The main function of the respiratory chain is to gradually transfer electrons from NADH and FADH2 (originating from the Krebs cycle) to oxygen (O2). With the addition of protons (H+), water molecules (H2O) are generated in Complex 4. NADH is a substrate or a coenzyme for the enzymatic activity of dehydrogenases that form part of the respiratory chain and reside in the inner membrane of the mitochondria. Further details on the biochemical properties of NADH can be found in publications by Chance and his collaborators.


Fig. 2.4
Available techniques for real time monitoring of energy metabolism at the tissue level. a Coupling between macrocirculation, monitored by pulse oximeter, and microcirculation. b–d Monitoring of cellular and intracellular compartments (see text for details). (© Reprinted with kind permission of Springer Science Business Media [83])
The formation of ATP depends on the sufficiency of substrate (i.e., glucose) and oxygen supply to the tissue by blood flow and hemoglobin oxygenation in the microcirculation (Fig. 2.4) as well as on the integrity of mitochondrial activity. Without a sufficient supply of ATP, cells cannot function properly and can ultimately die. Because most of the energy consumed by tissues is dependent upon the availability of oxygen, under normal mitochondrial function, there is high coupling between oxygen supply and energy production. When mitochondrial activity is damaged, for various reasons, this coupling is disrupted.
The electron transfer (oxidation/reduction) down the respiratory chain results in the production of adenosine triphosphate (ATP). Concomitantly with the electron transport, the respiratory chain components switch between reduced and oxidized states, each of which has different spectroscopic properties. Hydrolysis of the pyrophosphate bonds provides the energy necessary for the cell’s work. To assess this energy demand, it is necessary to measure different organ-specific parameters. For example, in the brain, the energy demand can be evaluated by measuring the extracellular levels of K+, which reflect the activity of the major ATP consumer, Na+/K+ATPase [2, 3]. In the heart, most of the energy is consumed by muscle contraction activity. On the other hand, the energy supply mechanism is the same for all tissues: oxygenated blood reaching the capillary bed releases O2 that diffuses into the cells. Therefore, it is possible to evaluate tissue energy supply by monitoring the following four parameters in all tissues: tissue blood flow (TBF), tissue blood volume (TBV), tissue oxyhemoglobin (HbO2), and mitochondrial NADH redox state (see Fig. 2.4).
The production of available energy (ATP) depends on pO2 (partial oxygen pressure) in the various compartments of the tissue. Information regarding pO2 in the tissue, therefore, is helpful for the evaluation of tissue metabolic activity. Oxygen levels can be measured by oxygen electrodes; however, the information collected is an average of the compartments around the electrode. In the past, the sensitivity and accuracy of oxygen electrodes in the range of 1 mm Hg (intracellular level) was not sufficient for the evaluation of mitochondrial function. As of today, the new developed techniques could provide more accurate values of oxygen. Other recent published studies have suggested that intracellular oxygen levels are in a higher range [4–8].
The need for an intracellular pO2 indicator, as a physiological and biochemical parameter of living tissue, emerged more than 50 years ago. Mitochondria are the intracellular organelles that consume most of the oxygen. Therefore, the redox state of electron carriers in isolated mitochondria in vitro as well as in vivo as a function of oxygen concentration has been extensively studied. Chance et al. concluded that “For a system at equilibrium, NADH is at the extreme low potential end of the chain, and this may be the oxygen indicator of choice in mitochondria and tissue as well” [9]. Lubbers in 1995 concluded that “the most important intrinsic luminescence indicator is NADH, an enzyme of which the reaction is connected with tissue respiration and energy metabolism” [10].
Currently, the ability to measure tissue energy metabolism at the microcirculation and cellular level is not available for clinical applications although a device has been developed.
2.2 Evaluation of Mitochondrial Function In Vivo
To monitor mitochondrial function, few options were used in the 1950s. As mentioned earlier, the activity of the electron transport chain could be evaluated by measuring the oxidation–reduction state of NADH, flavines (Fp), or the cytochromes. The classical table published by Chance and Williams in 1956 [11], as seen in Fig. 3.1, presents the effects of mitochondrial metabolic state on the oxidation–reduction of the various components active in the electron transport process. As discussed by Chance et al. [12], the most appropriate mitochondrial component to be measured was NADH. Nevertheless, in many studies, the flavoprotein (Fp) fluorescence was monitored either alone or together with NADH. Several papers described the technology enabling the monitoring of flavoproteins [13–16]. Isolated mitochondria from various organs such as heart [17, 18], brain, muscle, kidney, and liver [19] were monitored. The flying spot technique, for in vivo measurements, was developed and enabled the monitoring of Fp from animal brains [20–22] and from the human brain in vivo [23]. Over the years, various organs were monitored in vitro including the heart [24–26], muscle [27–29], liver [30], pancreatic island cells [31], and rabbit cornea [32].
In a large number of studies, Fp was monitored together with NADH after freezing the tissue in liquid nitrogen. The principles of the technique and its applications are presented in Sect. 6.6. This technique was applied mainly to study the brain of rats and gerbils [33–37] as well as liver of animals [38, 39] and human liver [40, 41].
Another use and application of Fp fluorescence in mapping brain activity was initiated in 2003 by Shibuki et al [42]. This group in Japan published at least another ten papers [43–52]. A second group started to use the same technology in the U.S. in 2004 and published a few papers [53–57]. A third group published a few more papers using the same technology in brain mapping [58–61].
A very interesting approach to monitor NADH and Fp simultaneously was developed by Masha Ranji and her group in Wisconsin. They developed the technology and used various organs in vitro (heart, kidney and lung) and in low temperature [62–74]. It seems that this group did not apply the technology to in vivo studies.
Immediately after the development of the fiber optic-based NADH fluorometry in 1972, I tried to extend the technology to enable the measurement of NADH together with Fp in the brain in vivo. Prof. Britton Chance told me that it would be very hard to obtain reliable results of Fp fluorescence because of the artifacts that originate from the large volume of blood in the brain. Nevertheless, we developed the two-channel time-sharing fluorometer–reflectometer for the measurements of two fluorescence and two reflectance signals from the same tissue volume [75].
The time-sharing fluorometer–reflectometer developed by Chance et al. [18] was used to measure NADH and Fp from the surface of the brain of an awake rat. Figure 2.5a shows a schematic presentation of the measuring system. The light source for the instrument was a 100 W water-cooled short mercury arc with a regulated power supply and automatic starter. The time-sharing filter is located in a disk driven by an air jet. The rotating disk includes the excitation and emission filters for NADH and flavoproteins. A partly silvered mirror (10 %) reflects the excitation light onto a second photomultiplier (P.M. 2), which is operated at a sufficiently low sensitivity so that components of the emission wavelengths are not detected. A 366-nm wavelength was used for excitation, and 450 nm was the appropriate wavelength for fluorescence emission measurement of NADH. The flavoproteins were excited at 460 nm, and the emitted wavelength was 560–580 nm.


Fig. 2.5
a Schematic presentation of time-sharing fluorometer-reflectometer connected to the surface of the brain by a light guide through a Plexiglas cannula. b Effect of anoxia (100 % N2) on the oxidation-reduction state of NADH, Fp, and ECoG (electrocortical activity). Time is running from left to right. The details of ECoG are shown in the left and right edges of the figure. (©Reprinted with kind permission of Springer Science + Business Media [75])
The calibration of the fluorescence and reflectance signals was accomplished while the brain was in the normoxic state by adjusting the photomultiplier dynode voltage to a point where the signals equaled the standard of 1 V [18].
The coupling of the fluorometer to the brain was accomplished by using a fused light guide made of quartz fibers (Schott, Mainz, Germany). The common part of the Y-shaped light guide has a 4-mm outer diameter and a 2-mm diameter of fibers.
To measure Fp fluorescence from the surface of the cortex, only animals with a clear and relatively blood-free area of the brain were used. Through this selection, it was possible to get a reasonable signal-to-noise ratio in about 50 % of the animals that were operated.
Figure 2.5b shows a typical response of the NADH and Fp to N2 exposure. The upper three traces show the measurement of NADH; the following three traces show the Fp measurement; and the bottom two traces show the electrocorticogram (ECoG) response to anoxia. To compensate and correct the NADH fluorescence measurement for hemodynamic alteration or swelling of the tissue, the ‘subtraction method’ described by Aubert et al. [76], Jobsis et al. [77], and Harbig et al. [78] was used. The Fp measurement shows as well that the uncorrected trace is affected by several factors. Therefore, the 460-nm reflectance was subtracted from the 577-nm fluorescence using the same technique as for NADH. The correction ratio for both NADH and Fp was 1:1 in all the animals.
The pattern of changes in the 450-nm fluorescence and 366-nm reflectance was similar to those previously described by us [79, 80]. After exposure to N2, the fluorescence of NADH increased and reached a plateau. When subsequently air was again supplied to the rat, a fast reoxidation of NADH (corrected trace) was recorded. The recovery of the uncorrected fluorescence and the reflectance traces to the base line occurred in 4–5 min. The uncorrected trace of the Fp shows a decrease in fluorescence (increase in reduced Fp) as a response to N2. The 460-nm reflectance show a small increase from N2. However, after air was given, a large decrease was recorded, which also affected the recovery of the 577-nm fluorescence. The subtraction of the 460-nm reflectance eliminated this effect, and the corrected fluorescence of Fp has the same kinetic changes as that of NADH.
The use of the fluorescence technique to measure NADH together with Fp from the surface of the brain of an awake animal was described in 1976 for the first time [75]. This type of measurement, using the time-sharing fluorometer, was accomplished previously only in a blood-free perfused system, as described by Scholz et al. [30] for the liver and by Chance et al. for the heart [81]. Two main problems arose when Fp fluorescence was measured from the surface of the brain in vivo. First, the presence of blood in the tissue causes the Fp fluorescence signal to be very small. Therefore, animals with a clear and blood-free exposed area of the brain were selected. Use of a 10-s response time of the fluorometer detectors gave a smooth and clear trace (Fig. 2.5b). The second problem was the lack of information concerning the correction for hemodynamic changes, hemoglobin oxygenation, or tissue swelling when measuring Fp fluorescence. The practical solution in these experiments was to use the 460-nm reflectance signal for correcting the Fp fluorescence (577 nm fluorescence). Subtraction of these two signals at a 1:1 ratio gave a good correction, similar to that achieved in the NADH measurement. Indirect evidence for the validity of the correction of Fp measurement is found in the fact that the responses of the Fp and NADH corrected traces to N2–air breathing cycles are kinetically similar. In various animals it was found that even when the uncorrected trace showed variation under N2 exposure, the kinetics of the corrected trace were similar.
When the same brain was exposed to cortical spreading depression, the NADH showed a clear oxidation cycle although the Fp showed a more reduced state of the Fp. This discrepancy between the NADH and the Fp responses indicated to us that more research is needed before a good Fp signal will be “clean” from artifacts. To further demonstrate and prove that the fluorescence signal measured by our device is of mitochondrial origin (Fig. 2.5), we performed another animal study. We used a partial ischemic brain model developed under 24 h of bilateral carotid arteries occlusion in the rat. Oscillations in the level of NADH caused by ischemia was recorded, as shown in detail in Sect 7.2.6. We used the same model in which NADH and Fp were monitored together. We verified that the oscillations in brain NADH signals are mitochondrial. To do so, we monitored simultaneously the fluorescence of NADH and Fp. The appearance of oscillations in the oxidized Fp fluorescence (only mitochondrial) can be seen in Fig. 2.6 (carotid arteries were occluded for 24 h). The effect of anoxia (Fig. 2.6a) as well as of Spreading Depression (SD) (Fig. 2.6b) can be seen. NADH was monitored simultaneously with the Fp as well as two reflectance signals (366 and 460 nm) using a time-sharing fluorometer [75]. Because of the difference in fluorescent properties, NADH increased during anoxia whereas Fp decreased, but both changes have similar kinetics, and the brain respiratory chain became more reduced. During SD both signals (NADH and Fp) showed an increase in redox state because of the limited amounts of oxygen available in the ischemic brain [82]. Under both anoxia and SD, the oscillations disappeared during the perturbation itself.


Fig. 2.6
Effects of anoxia (a) and spreading depression (b) on oscillations in NADH and Fp fluorescence NADH reflectance. The records were obtained from a rat with bilateral carotid arteries occluded for 24 h: channels one to three represent NADH measurement; Fp fluorescence and reflectance are shown in channels four to six. (© Maney Publishing, reprinted with permission [85])
In conclusion, we have good reason to believe that Fp could be monitored from the regular brain correctly only when a good correction technique for changes in blood volume and hemoglobin oxygenation is developed.
References
1.
Barcroft J (1914) The respiratory function of blood. Cambridge University Press, CambridgeCrossRef
2.
Mayevsky A (1984) Brain NADH redox state monitored in vivo by fiber optic surface fluorometry. Brain Res Rev 7:49–68CrossRef
3.
4.
Pittman RN (2011) Oxygen gradients in the microcirculation. Acta Physiol 202:311–322. doi:10.1111/j.1748-1716.2010.02232.x CrossRef
5.
Schober P, Schwarte LA (2012) From system to organ to cell: oxygenation and perfusion measurement in anesthesia and critical care. J Clin Monit Comput 26:255–265. doi:10.1007/s10877-012-9350-4 PubMedCentralPubMedCrossRef
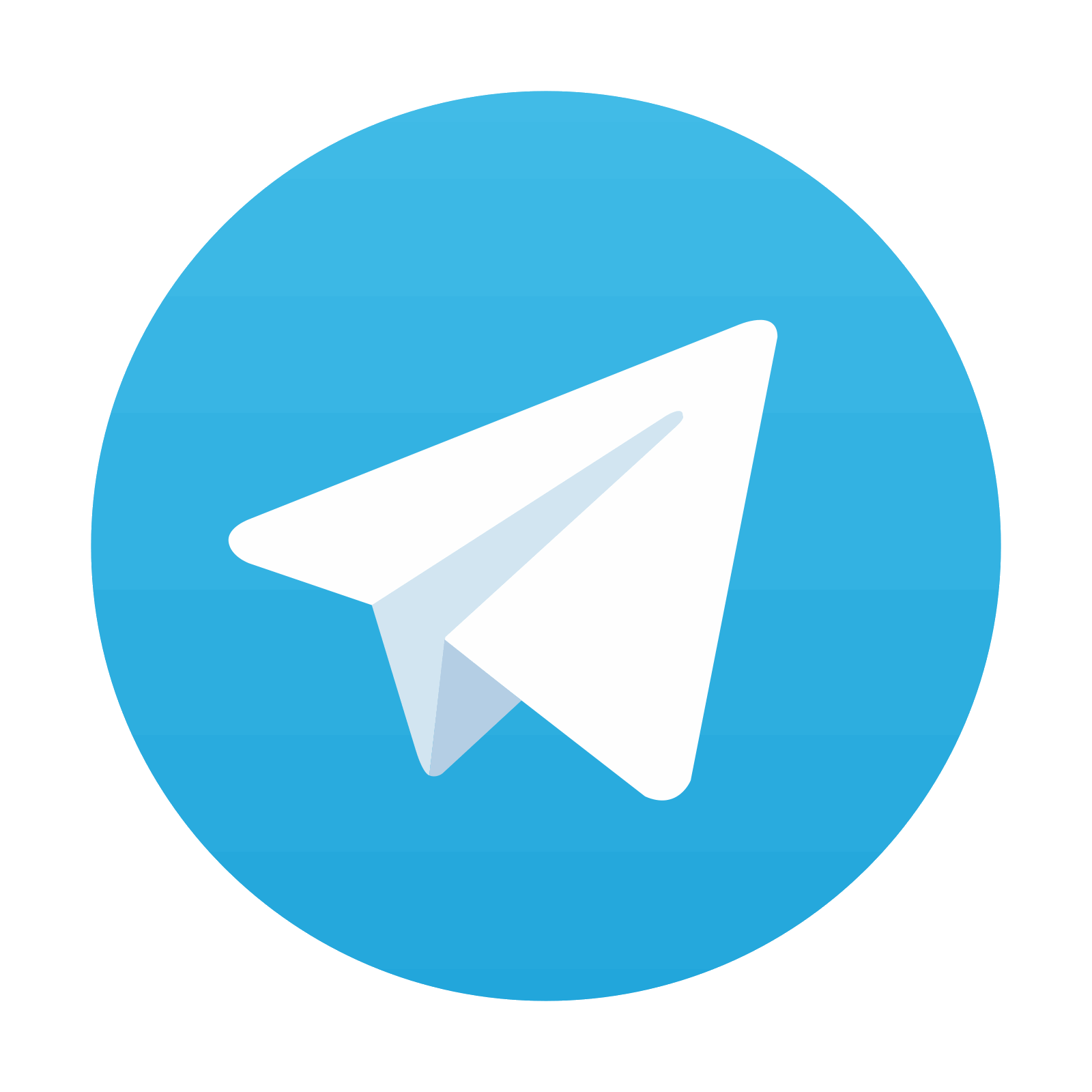
Stay updated, free articles. Join our Telegram channel
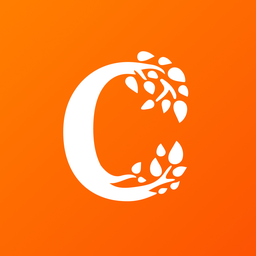
Full access? Get Clinical Tree
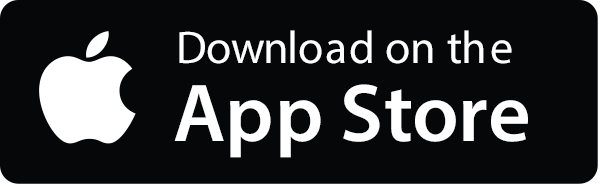
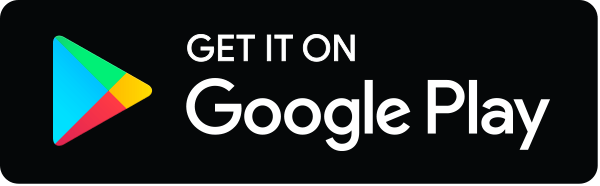
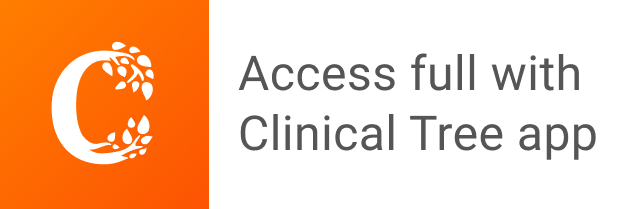