Fatty acids are synthesized mainly in the liver in humans, with dietary glucose serving as the major source of carbon. Glucose is converted through glycolysis to pyruvate, which enters the mitochondrion and forms both acetyl coenzyme A (acetyl CoA) and oxaloacetate (Fig. 31.1). These two compounds condense, forming citrate. Citrate is transported to the cytosol, where it is cleaved to form acetyl CoA, the source of carbon for the reactions that occur on the fatty acid synthase complex. The key regulatory enzyme for the process, acetyl CoA carboxylase, produces malonyl CoA from acetyl CoA.
FIGURE 31.1 Lipogenesis, the synthesis of triacylglycerols from glucose. In humans, the synthesis of fatty acids from glucose occurs mainly in the liver. Fatty acids (FA) are converted to triacylglycerols (TG), packaged in very-low-density lipoprotein (VLDL), and secreted into the blood. CoA, coenzyme A; DHAP, dihydroxyacetone phosphate; FACoA, fatty acyl coenzyme A; Glycerol 3-P, glycerol 3-phosphate; NADP, nicotinamide adenine dinucleotide phosphate; OAA, oxaloacetate.
The growing fatty acid chain, attached to the fatty acid synthase complex in the cytosol, is elongated by the sequential addition of two-carbon units provided by malonyl CoA. NADPH, produced by the pentose phosphate pathway and the malic enzyme, provides reducing equivalents. When the growing fatty acid chain is 16 carbons in length, it is released as palmitate. After activation to a CoA derivative, palmitate can be elongated and desaturated to produce a series of fatty acids.
Fatty acids, produced in cells or obtained from the diet, are used by various tissues for the synthesis of triacylglycerols (the major storage form of fuel) and the glycerophospholipids and sphingolipids (the major components of cell membranes).
In the liver, triacylglycerols are produced from fatty acyl CoA and glycerol 3-phosphate. Phosphatidic acid serves as an intermediate in this pathway. The triacylglycerols are not stored in the liver but rather packaged with apolipoproteins and other lipids in very-low-density lipoprotein (VLDL) and secreted into the blood (see Fig. 31.1).
In the capillaries of various tissues (particularly adipose tissue, muscle, and the lactating mammary gland), lipoprotein lipase (LPL) digests the triacylglycerols of VLDL, forming fatty acids and glycerol (Fig. 31.2). The glycerol travels to the liver where it is used. Some of the fatty acids are oxidized by muscle and other tissues. After a meal, however, most of the fatty acids are converted to triacylglycerols in adipose cells, where they are stored. These fatty acids are released during fasting and serve as the predominant fuel for the body.
FIGURE 31.2 Fate of very-low-density lipoprotein (VLDL) triacylglycerol (TG). The TG of VLDL, produced in the liver, is digested by lipoprotein lipase (LPL) present on the endothelial cells lining the capillaries in adipose and skeletal muscle tissue. Fatty acids are released and either oxidized in the muscle or stored in adipose tissue as TG. Glycerol is used by the liver because hepatocytes exclusively contain glycerol kinase. FA, fatty acid (or fatty acyl group); FACoA, fatty acyl coenzyme A.
Glycerophospholipids are also synthesized from fatty acyl CoA, which forms esters with glycerol 3-phosphate, producing phosphatidic acid. Various head groups are added to carbon 3 of the glycerol 3-phosphate moiety of phosphatidic acid, generating amphipathic compounds such as phosphatidylcholine, phosphatidylinositol, and cardiolipin (Fig. 31.3A). In the formation of plasmalogens and platelet-activating factor (PAF), a long-chain fatty alcohol forms an ether with carbon 1, replacing the fatty acyl ester (see Fig. 31.3B). Cleavage of phospholipids is catalyzed by phospholipases found in cell membranes, lysosomes, and pancreatic juice.
FIGURE 31.3 A. General structure of a glycerophospholipid. The fatty acids are joined by ester bonds to the glycerol moiety. Various combinations of fatty acids may be present. The fatty acid at carbon 2 of the glycerol is usually unsaturated. The head group is the group attached to the phosphate on position 3 of the glycerol moiety. The most common head group is choline, but ethanolamine, serine, inositol, or phosphatidylglycerol also may be present. The phosphate group is negatively charged, and the head group may carry a positive charge (choline and ethanolamine), or both a positive and a negative charge (serine). The inositol may be phosphorylated and thus negatively charged. B. General structure of a plasmalogen. Carbon 1 of glycerol is joined to a long-chain fatty alcohol by an ether linkage. The fatty alcohol group has a double bond between carbons 1 and 2. The head group is usually ethanolamine or choline. C. General structures of the sphingolipids. The “backbone” is sphingosine rather than glycerol. Ceramide is sphingosine with a fatty acid joined to its amino group by an amide linkage. Sphingomyelin contains phosphocholine, whereas glycolipids (D) contain carbohydrate groups.
Sphingolipids, which are prevalent in membranes and the myelin sheath of the central nervous system, are built on serine rather than glycerol. In the synthesis of sphingolipids, serine and palmityol CoA condense, forming a compound that is related to sphingosine. Reduction of this compound, followed by the addition of a second fatty acid in amide linkage, produces ceramide. Carbohydrate groups attach to ceramide, forming glycolipids such as the cerebrosides, globosides, and gangliosides (see Fig. 31.3D). The addition of phosphocholine to ceramide produces sphingomyelin (see Fig. 31.3C). These sphingolipids are degraded by lysosomal enzymes.
THE WAITING ROOM 
Emma W. has done well with regard to her respiratory function since her earlier hospitalization for an acute asthma exacerbation (see Chapter 28). She has been maintained on two puffs of triamcinolone acetonide, a potent inhaled corticosteroid, two times per day, and has not required systemic steroids for months. The glucose intolerance precipitated by high intravenous and oral doses of the synthetic glucocorticoid prednisone during her earlier hospitalization resolved after this drug was discontinued. She has come to her doctor now because she is concerned that the low-grade fever and cough she has developed over the last 36 hours may trigger another asthma exacerbation.
Percy V.’s mental depression slowly responded to antidepressant medication, to the therapy sessions with his psychiatrist, and to frequent visits from an old high school sweetheart whose husband had died several years earlier. While he was hospitalized for malnutrition, Percy V.’s appetite returned. By the time of discharge, he had gained back 8 of the 22 lb he had lost and weighed 131 lb.
During the next few months, Percy V. developed a craving for “sweet foods” such as the candy he bought and shared with his new friend. After 6 months of this high-carbohydrate courtship, Percy V. had gained another 22 lb and now weighed 155 lb, which was 8 lb more than he weighed when his depression began. He became concerned about the possibility that he would soon be overweight and consulted his dietitian, explaining that he had faithfully followed his low-fat diet but had “gone overboard” with carbohydrates. He asked whether it was possible to become fat without eating fat.
Cora N.’s hypertension and systolic heart failure have been well controlled on medication, and she has lost 10 lb since she had her recent heart attack. Her fasting serum lipid profile before discharge from the hospital indicated a significantly elevated serum low-density lipoprotein (LDL) cholesterol level of 175 mg/dL, a serum triacylglycerol level of 280 mg/dL (reference range, 60 to 150 mg/dL), and a serum high-density lipoprotein (HDL) cholesterol level of 34 mg/dL (reference range >50 mg/dL for healthy women). While she was still in the hospital, she was asked to obtain the most recent serum lipid profiles of her older brother and her younger sister, both of whom were experiencing chest pain. Her brother’s profile showed normal triacylglycerols, moderately elevated LDL cholesterol, and significantly suppressed HDL cholesterol levels. Her sister’s profile showed only hypertriglyceridemia (high blood triacylglycerols).
Christy L. was born 9 weeks prematurely. She appeared normal until about 30 minutes after delivery when her respirations became rapid at 64 breaths per minute with audible respiratory grunting. The spaces between her ribs (intercostal spaces) retracted inward with each inspiration, and her lips and fingers became cyanotic from a lack of oxygen in her arterial blood. An arterial blood sample indicated a low partial pressure of oxygen (PO2) and a slightly elevated partial pressure of carbon dioxide (PCO2). The arterial pH was somewhat suppressed, in part from an accumulation of lactic acid secondary to the hypoxemia (a low level of oxygen in her blood). A chest radiograph showed a fine reticular granularity of the lung tissue, especially in the left lower lobe area. From these clinical data, a diagnosis of respiratory distress syndrome (RDS) (formally known as hyaline membrane disease) was made.
Christy L. was immediately transferred to the neonatal intensive care unit, where, with intensive therapy, she improved.
I. Fatty Acid Synthesis
Fatty acids are synthesized whenever an excess of calories is ingested. The major source of carbon for the synthesis of fatty acids is dietary carbohydrate. An excess of dietary protein also can result in an increase in fatty acid synthesis. In this case, the carbon source is amino acids that can be converted to acetyl CoA or tricarboxylic acid (TCA) cycle intermediates (see Chapter 38). Fatty acid synthesis occurs primarily in the liver in humans, although it can also occur, to a lesser extent, in adipose tissue.
When an excess of dietary carbohydrate is consumed, glucose is converted to acetyl CoA, which provides the two-carbon units that condense in a series of reactions on the fatty acid synthase complex, producing palmitate (see Fig. 31.1). Palmitate is then converted to other fatty acids. The fatty acid synthase complex is located in the cytosol and, therefore, it uses cytosolic acetyl CoA.
A. Conversion of Glucose to Cytosolic Acetyl Coenzyme A
The pathway for the synthesis of cytosolic acetyl CoA from glucose begins with glycolysis, which converts glucose to pyruvate in the cytosol (Fig. 31.4). Pyruvate enters mitochondria, where it is converted to acetyl CoA by pyruvate dehydrogenase and to oxaloacetate by pyruvate carboxylase. The pathway pyruvate follows is dictated by the acetyl CoA levels in the mitochondria. When acetyl CoA levels are high, pyruvate dehydrogenase is inhibited and pyruvate carboxylase activity is stimulated. As oxaloacetate levels increase because of the activity of pyruvate carboxylase, oxaloacetate condenses with acetyl CoA to form citrate. This condensation reduces the acetyl CoA levels, which leads to the activation of pyruvate dehydrogenase and inhibition of pyruvate carboxylase. Through such reciprocal regulation, citrate can be continuously synthesized and transported across the inner mitochondrial membrane. In the cytosol, citrate is cleaved by citrate lyase to re-form acetyl CoA and oxaloacetate. This circuitous route is required because pyruvate dehydrogenase, the enzyme that converts pyruvate to acetyl CoA, is found only in mitochondria and because acetyl CoA cannot directly cross the mitochondrial membrane.
FIGURE 31.4 Conversion of glucose to cytosolic acetyl coenzyme A (acetyl CoA) and the fate of citrate in the cytosol. Citrate lyase is also called citrate cleavage enzyme. ADP, adenosine diphosphate; ATP, adenosine triphosphate; NAD, nicotinamide adenine dinucleotide; OAA, oxaloacetate; Pi, inorganic phosphate; ↑, inducible enzyme.
Reduced nicotinic adenine dinucleotide phosphate (NADPH) is required for fatty acid synthesis and is generated by the pentose phosphate pathway (see Chapter 27) and from recycling of the oxaloacetate produced by citrate lyase (see Fig. 31.4). Oxaloacetate is converted back to pyruvate in two steps: the reduction of oxaloacetate to malate by the NAD+-dependent malate dehydrogenase and the oxidation and decarboxylation of malate to pyruvate by an NADP+-dependent malate dehydrogenase (malic enzyme) (Fig. 31.5). The pyruvate formed by malic enzyme is reconverted to citrate. The NADPH that is generated by malic enzyme, along with the NADPH generated by glucose 6-phosphate dehydrogenase and gluconate 6-phosphate dehydrogenase in the pentose phosphate pathway, is used for the reduction reactions that occur on the fatty acid synthase complex.
FIGURE 31.5 Reaction catalyzed by malic enzyme. This enzyme is also called the decarboxylating or nicotinamide adenine dinucleotide phosphate (NADP)-dependent malate dehydrogenase.
The generation of cytosolic acetyl CoA from pyruvate is stimulated by elevation of the insulin/glucagon ratio after a carbohydrate meal. Insulin activates pyruvate dehydrogenase by stimulating the phosphatase that dephosphorylates the enzyme to an active form (see Chapter 23). The synthesis of malic enzyme, glucose 6-phosphate dehydrogenase, and citrate lyase is induced by the high insulin/glucagon ratio. The ability of citrate to accumulate and to leave the mitochondrial matrix for the synthesis of fatty acids is attributable to the allosteric inhibition of isocitrate dehydrogenase by high energy levels within the matrix under these conditions. The concerted regulation of glycolysis and fatty acid synthesis is described in Chapter 34.
B. Conversion of Acetyl CoA to Malonyl CoA
Cytosolic acetyl CoA is converted to malonyl CoA, which serves as the immediate donor of the two-carbon units that are added to the growing fatty acid chain on the fatty acid synthase complex. To synthesize malonyl CoA, acetyl CoA carboxylase adds a carboxyl group to acetyl CoA in a reaction that requires biotin and adenosine triphosphate (ATP) (Fig. 31.6).
FIGURE 31.6 Reaction catalyzed by acetyl coenzyme A (acetyl CoA) carboxylase. Initially, CO2 is covalently attached to biotin, which is linked by an amide bond to the ε-amino group of a lysine residue of the enzyme. Hydrolysis of adenosine triphosphate (ATP) is required for the attachment of CO2 to biotin. Subsequently, the CO2 is transferred to acetyl CoA to form malonyl CoA. CoA, coenzyme A; Pi, inorganic phosphate.
Acetyl CoA carboxylase is the rate-limiting enzyme of fatty acid synthesis. Its activity is regulated by phosphorylation, allosteric modification, and induction/repression of its synthesis (Fig. 31.7). Citrate allosterically activates acetyl CoA carboxylase by causing the individual enzyme molecules (each composed of four subunits) to polymerize. Palmitoyl CoA, produced from palmitate (the end product of fatty acid synthase activity), inhibits acetyl CoA carboxylase. Phosphorylation by the AMP-activated protein kinase inhibits the enzyme in the fasting state when energy levels are low. Acetyl CoA carboxylase is activated by dephosphorylation in the fed state when energy and insulin levels are high. A high insulin/glucagon ratio also results in induction of the synthesis of both acetyl CoA carboxylase and the next enzyme in the pathway, fatty acid synthase.
FIGURE 31.7 Regulation of acetyl coenzyme A (acetyl CoA) carboxylase. This enzyme is regulated allosterically, both positively and negatively, by phosphorylation () and dephosphorylation, and by diet-induced induction (↑). It is active in the dephosphorylated state when citrate causes it to polymerize. Dephosphorylation is catalyzed by an insulin-stimulated phosphatase. Low energy levels, via activation of the adenosine monophosphate (AMP)-activated protein kinase, cause the enzyme to be phosphorylated and inactivated. The ultimate product of fatty acid synthesis, palmitate, is converted to its coenzyme A (CoA) derivative, palmitoyl coenzyme A (palmitoyl CoA), which inhibits the enzyme. A high-calorie diet increases the rate of transcription of the gene for acetyl CoA carboxylase, whereas a low-calorie diet reduces transcription of this gene. ADP, adenosine diphosphate; ATP, adenosine triphosphate; Pi, inorganic phosphate.
C. Fatty Acid Synthase Complex
As an overview, fatty acid synthase sequentially adds two-carbon units from malonyl CoA to the growing fatty acyl chain to form palmitate. After the addition of each two-carbon unit, the growing chain undergoes two reduction reactions that require NADPH.
Fatty acid synthase is a large enzyme composed of two identical subunits, which each have seven catalytic activities and an acyl carrier protein (ACP) segment in a continuous polypeptide chain. The ACP segment contains a phosphopantetheine residue that is derived from the cleavage of coenzyme A. The key feature of the ACP is that it contains a free sulfhydryl group (from the phosphopantetheine residue). The two dimers associate in a head-to-tail arrangement so that the phosphopantetheinyl sulfhydryl group on one subunit and a cysteinyl sulfhydryl group on another subunit are closely aligned.
In the initial step of fatty acid synthesis, an acetyl moiety is transferred from acetyl CoA to the ACP phosphopantetheinyl sulfhydryl group of one subunit and then to the cysteinyl sulfhydryl group of the other subunit. The malonyl moiety from malonyl CoA then attaches to the ACP phosphopantetheinyl sulfhydryl group of the first subunit. The acetyl and malonyl moieties condense, with the release of the malonyl carboxyl group as CO2. A four-carbon β-keto acyl chain is now attached to the ACP phosphopantetheinyl sulfhydryl group (Fig. 31.8).
FIGURE 31.8 Addition of a two-carbon unit to an acetyl group on fatty acid synthase (FAS). The malonyl group attaches to the phosphopantetheinyl residue (P) of the acyl carrier protein (ACP) of the fatty acid synthase. The acetyl group, which is attached to a cysteinyl sulfhydryl group, condenses with the malonyl group. CO2 is released, and a 3-ketoacyl group is formed. The carbon that eventually forms the ω-methyl group of palmitate is labeled ω (and originates from acetyl CoA). CoA, coenzyme A; Malonyl CoA , malonyl coenzyme A.
A series of three reactions reduce the four-carbon keto group to an alcohol, then removes water to form a double bond, and finally reduces the double bond (Fig. 31.9). NADPH provides the reducing equivalents for these reactions. The net result is that the original acetyl group is elongated by two carbons.
FIGURE 31.9 Reduction of a β-ketoacyl group on the fatty acid synthase complex. Reduced nicotinamide adenine dinucleotide phosphate (NADPH) is the reducing agent.
The four-carbon fatty acyl chain is then transferred to the cysteinyl sulfhydryl group and subsequently condenses with a malonyl group. This sequence of reactions is repeated until the chain is 16 carbons in length. At this point, hydrolysis occurs, and palmitate is released (Fig. 31.10).
FIGURE 31.10 Synthesis of palmitate on the fatty acid synthase complex. Initially, acetyl coenzyme A (acetyl CoA) adds to the synthase. It provides the ω-methyl group of palmitate. Malonyl coenzyme A (Malonyl CoA) provides the two-carbon units that are added to the growing fatty acyl chain. The addition and reduction steps are repeated until palmitate is produced. (1) Transfer of the malonyl group to the phosphopantetheinyl residue. (2) Condensation of the malonyl and fatty acyl groups. (3) Reduction of the β-ketoacyl group. (4) Dehydration. (5) Reduction of the double bond. ADP, adenosine diphosphate; ATP, adenosine triphosphate; Cys-SH, a cysteinyl residue on a different subunit of the fatty acid synthase; NADP, nicotinamide adenine dinucleotide phosphate; P, a phosphopantetheinyl group attached to the fatty acid synthase complex; Pi, inorganic phosphate.
Palmitate is elongated and desaturated to produce a series of fatty acids. In the liver, palmitate and other newly synthesized fatty acids are converted to triacylglycerols that are packaged into VLDL for secretion.
In the liver, the oxidation of newly synthesized fatty acids back to acetyl CoA via the mitochondrial β-oxidation pathway is prevented by malonyl CoA. Carnitine palmitoyltransferase I, the enzyme involved in the transport of long-chain fatty acids into mitochondria (see Chapter 30), is inhibited by malonyl CoA (Fig. 31.11). Malonyl CoA levels are elevated when acetyl CoA carboxylase is activated, and thus fatty acid oxidation is inhibited while fatty acid synthesis is proceeding. This inhibition prevents the occurrence of a futile cycle.
FIGURE 31.11 Inhibition of carnitine palmitoyltransferase I (CPTI, also called carnitine acyltransferase I) by malonyl coenzyme A (malonyl CoA). During fatty acid (FA) synthesis, malonyl CoA levels are high. This compound inhibits CPTI, which is involved in the transport of long-chain fatty acids into mitochondria for β-oxidation. This mechanism prevents newly synthesized fatty acids from undergoing immediate oxidation. Acetyl CoA, acetyl coenzyme A; CPTII, carnitine palmitoyltransferase II.
D. Elongation of Fatty Acids
After synthesis on the fatty acid synthase complex, palmitate is activated, forming palmitoyl CoA. Palmitoyl CoA and other activated long-chain fatty acids can be elongated, two carbons at a time, by a series of reactions that occur in the endoplasmic reticulum (Fig. 31.12). Malonyl CoA serves as the donor of the two-carbon units, and NADPH provides the reducing equivalents. The series of elongation reactions resemble those of fatty acid synthesis except that the fatty acyl chain is attached to coenzyme A rather than to the phosphopantetheinyl residue of an ACP. The major elongation reaction that occurs in the body involves the conversion of palmityol CoA (C16) to stearyl CoA (C18). Very-long-chain fatty acids (C22 to C24) are also produced, particularly in the brain.
FIGURE 31.12 Elongation of long-chain fatty acids in the endoplasmic reticulum. The example shown is palmitoyl CoA being extended to stearoyl CoA. CoA, coenzyme A; Malonyl CoA, malonyl coenzyme A; NADP, nicotinamide adenine dinucleotide phosphate.
E. Desaturation of Fatty Acids
Desaturation of fatty acids involves a process that requires molecular oxygen (O2), NADH, and cytochrome b5. The reaction, which occurs in the endoplasmic reticulum, results in the oxidation of both the fatty acid and NADH (Fig. 31.13). The most common desaturation reactions involve the placement of a double bond between carbons 9 and 10 in the conversion of palmitic acid to palmitoleic acid (16:1, Δ9) and the conversion of stearic acid to oleic acid (18:1, Δ9). Other positions that can be desaturated in humans include carbons 5 and 6.
FIGURE 31.13 Desaturation of fatty acids. The process occurs in the endoplasmic reticulum and uses molecular oxygen. Both the fatty acid and reduced nicotinamide adenine dinucleotide (NADH) are oxidized. Human desaturases cannot introduce double bonds between carbon 9 and the methyl end and are limited to positions 5, 6, and 9. Therefore, m is ≤7. CoA, coenzyme A; cyt, cytochrome; FAD, flavin adenine dinucleotide; Fatty acyl CoA, fatty acyl coenzyme A.
Polyunsaturated fatty acids with double bonds three carbons from the methyl end (ω3 fatty acids) and six carbons from the methyl end (ω6 fatty acids) are required for the synthesis of eicosanoids (see Section II of this chapter). Because humans cannot synthesize these fatty acids de novo (i.e., from glucose via palmitate), they must be present in the diet or the diet must contain other fatty acids that can be converted to these fatty acids. We obtain ω6 and ω3 polyunsaturated fatty acids mainly from dietary plant oils that contain the ω6 fatty acid linoleic acid (18:2, Δ9,12) and the ω3 fatty acid α-linolenic acid (18:3, Δ9,12,15). Linoleic and linolenic acids are thus considered essential fatty acids for the human diet. In the body, linoleic acid can be converted by elongation and desaturation reactions to arachidonic acid (20:4, Δ5,8,11,14), which is used for the synthesis of the major class of human prostaglandins and other eicosanoids (Fig. 31.14). Elongation and desaturation of α-linolenic acid produce eicosapentaenoic acid (EPA; 20:5, Δ5,8,11,14,17), which is the precursor of a different class of eicosanoids (see Section II).
FIGURE 31.14 Conversion of linoleic acid to arachidonic acid. Dietary linoleic acid (as linoleoyl CoA) is desaturated at carbon 6, elongated by two carbons, and then desaturated at carbon 5 to produce arachidonyl CoA. CoA, coenzyme A; NAD, nicotinamide adenine dinucleotide.
Plants are able to introduce double bonds into fatty acids in the region between C10 and the ω-end and, therefore, can synthesize ω3 and ω6 polyunsaturated fatty acids. Fish oils also contain ω3 and ω6 fatty acids, particularly eicosapentaenoic acid (EPA; ω3, 20:5, Δ5,8,11,14,17) and docosahexaenoic acid (DHA; ω3, 22:6, Δ4,7,10,13,16,19). The fish obtain these fatty acids by eating phytoplankton (plants that float in water).
Arachidonic acid is listed in some textbooks as an essential fatty acid. Although it is an ω6 fatty acid, it is not essential in the diet if linoleic acid is present because arachidonic acid can be synthesized from dietary linoleic acid (see Fig. 31.14).
The essential fatty acid linoleic acid is required in the diet for at least three reasons. (1) It serves as a precursor of arachidonic acid from which eicosanoids are produced. (2) It covalently binds another fatty acid attached to cerebrosides in the skin, forming an unusual lipid (acylglucosylceramide) that helps to make the skin impermeable to water. This function of linoleic acid may help to explain the red, scaly dermatitis and other skin problems associated with a dietary deficiency of essential fatty acids. (3) It is the precursor of important neuronal fatty acids.
II. Synthesis of the Eicosanoids
Eicosanoids (“eicosa” is the Greek word for the number 20) are biologically active lipids, derived from 20 carbon fatty acids. They consist primarily of the prostaglandins, thromboxanes, and leukotrienes. These lipids are the most potent regulators of cellular function in nature and are produced by almost every cell in the body. They act mainly as “local” hormones, affecting the cells that produce them or neighboring cells of a different type.
Eicosanoids participate in many processes in the body, particularly the inflammatory response that occurs after infection or injury. The inflammatory response is the sum of the body’s efforts to destroy invading organisms and to repair damage. It includes control of bleeding through the formation of blood clots. In the process of protecting the body from a variety of insults, the inflammatory response can produce symptoms such as pain, swelling, and fever. An exaggerated or inappropriate expression of the normal inflammatory response may occur in individuals who have allergic or hypersensitivity reactions.
In addition to participating in the inflammatory response, eicosanoids also regulate smooth muscle contraction (particularly in the intestine and uterus). They increase water and sodium excretion by the kidney and are involved in regulating blood pressure. They frequently serve as modulators; some eicosanoids stimulate and others inhibit the same process. For example, some serve as constrictors and others as dilators of blood vessels. They are also involved in regulating bronchoconstriction and bronchodilation.
A. Source of the Eicosanoids
The most abundant, and therefore the most common precursor of the eicosanoids is arachidonic acid (C20:4, Δ5, 8, 11, 14), a polyunsaturated fatty acid with 20 carbons and four double bonds. Because arachidonic acid cannot be synthesized in the body (it is an ω-6 fatty acid), the diet must contain arachidonic acid or other fatty acids from which arachidonic acid can be produced (such as linoleic acid, found in plant oils). An overview of eicosanoid biosynthesis is shown in Figure 31.15.
FIGURE 31.15 Overview of eicosanoid metabolism. Eicosanoids are produced from fatty acids released from membrane phospholipids. In humans, arachidonic acid is the major precursor of the eicosanoids, which include the prostaglandins (PG), leukotrienes (LT), and thromboxanes (TX). ⊝ “inhibits”; cyt, cytochrome; NSAID, nonsteroidal anti-inflammatory drug.
The arachidonic acid present in membrane phospholipids is released from the lipid bilayer as a consequence of the activation of membrane-bound phospholipase A2 or C (see Fig. 31.15). This activation occurs when a variety of stimuli (agonists), such as histamine or the cytokines interact with a specific plasma membrane receptor on the target cell surface. Phospholipase A2 is specific for the sn-2 position of phosphoacylglycerols, the site of attachment of arachidonic acid to the glycerol moiety. Phospholipase C, conversely, hydrolyzes phosphorylated inositol from the inositol glycerophospholipids, generating a diacylglycerol containing arachidonic acid. This arachidonic acid is subsequently released by the action of other lipases.
B. Pathways for Eicosanoid Synthesis
After arachidonic acid is released into the cytosol, it is converted to eicosanoids by a variety of enzymes with activities that vary among tissues. This variation explains why some cells, such as those in the vascular endothelium, synthesize prostaglandins E2 and I2 (PGE2 and PGI2), whereas cells such as platelets synthesize primarily thromboxane A2 (TXA2) and 12-hydroxyeicosatetraenoic acid (12-HETE).
Three major pathways for the metabolism of arachidonic acid occur in various tissues (Fig. 31.16). The first of these, the cyclooxygenase pathway, leads to the synthesis of prostaglandins and thromboxanes. The second, the lipoxygenase pathway, yields the leukotrienes, HETEs, and lipoxins. The third pathway, catalyzed by the cytochrome P450 system, is responsible for the synthesis of the epoxides, HETEs, and diHETEs. Only the cyclooxygenase pathway will be discussed further in this text. Information about the other pathways can be found in the Chapter 31 online supplement to the text.
FIGURE 31.16 Pathways for the metabolism of arachidonic acid. diHETE, dihydroxyeicosatetraenoic acid; HETE, hydroxyeicosatetraenoic acid; HPETE, hydroperoxyeicosatetraenoic acid; PG, prostaglandin.
C. Cyclooxygenase Pathway: Synthesis of the Prostaglandins and Thromboxanes
1. Structures of the Prostaglandins
Prostaglandins are fatty acids containing 20 carbon atoms, including an internal 5-carbon ring. In addition to this ring, each of the biologically active prostaglandins has a hydroxyl group at carbon 15, a double bond between carbons 13 and 14, and various substituents on the ring.
The nomenclature for the prostaglandins (PGs) involves the assignment of a capital letter (PGE), an Arabic numeral subscript (PGE1), and, for the PGF family, a Greek letter subscript (e.g., PGF2α). The capital letter, in this case, “F,” refers to the ring substituents shown in Figure 31.17.
FIGURE 31.17 Ring substituents of the prostaglandins (PG). The letter after PG denotes the configuration of the ring and its substituents. R4, R7, and R8 represent the nonring portions of the molecule. R4 contains four carbons (including the carboxyl group). R7 and R8 contain seven and eight carbons, respectively. Note that the prostacyclins (PGI) contain two rings.
The subscript that follows the capital letter (PGF1) refers to the PG series 1, 2, or 3, determined by the number of unsaturated bonds present in the linear portion of the hydrocarbon chain. It does not include double bonds in the internal ring. Prostaglandins of the 1-series have one double bond (between carbons 13 and 14). The 2-series has two double bonds (between carbons 13 and 14, and 5 and 6), and the 3-series has three double bonds (between carbons 13 and 14, 5 and 6, and 17 and 18). The double bonds between carbons 13 and 14 are trans; the others are cis. The precursor for the 1-series of prostaglandins is cis Δ8,11,14 eicosatrienoic acid; for the 2-series, it is arachidonic acid; for the 3-series, it is cis Δ5,8,11,14,17 eicosapentaenoic acid.
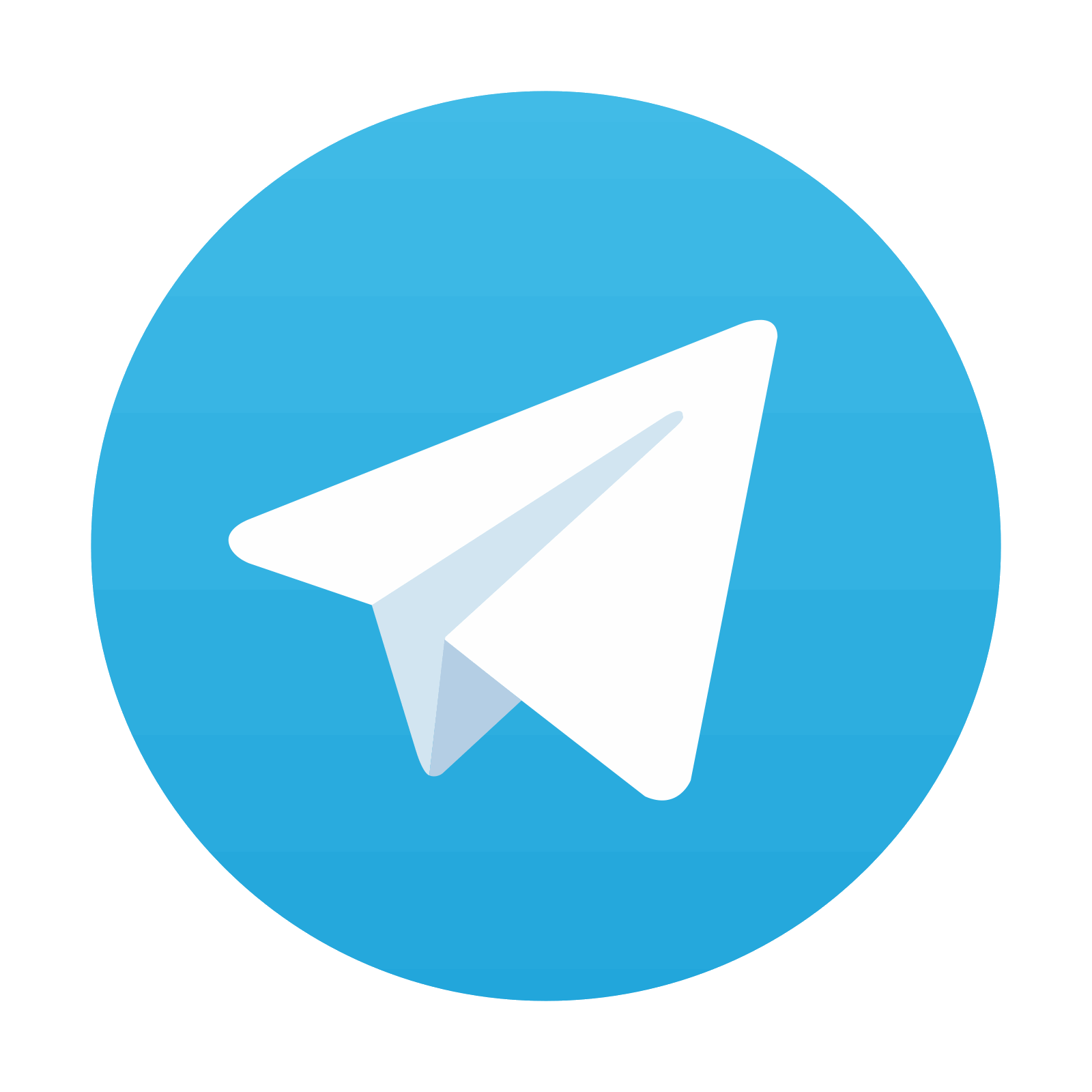
Stay updated, free articles. Join our Telegram channel
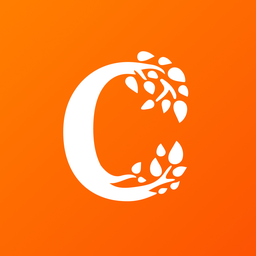
Full access? Get Clinical Tree
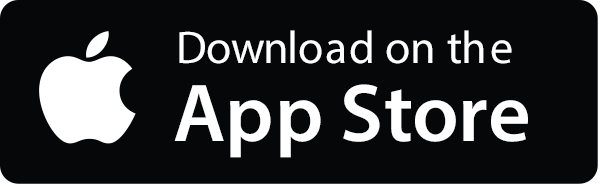
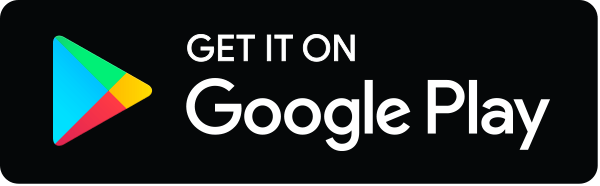