Human genetics is defined as the study of heredity and the variation in inherited characteristics, as they apply to humans. As discussed in Chapter 11, human DNA is spread across 46 chromosomes (23 inherited from the mother, and 23 inherited from the father). The autosomal chromosomes are numbered from 1 through 22, originally based on size (numbered from largest to smallest, with 1 being the largest and 22 the smallest, although recent data indicate that 21 is smaller than 22), and then X or Y, which are referred to as the sex chromosomes. Human somatic cells are diploid, with two copies of each autosomal chromosome (one from each parent) and two sex chromosomes—XX will be a female, XY a male. Women transmit their X chromosome to their children, while the father transmits either an X or a Y chromosome.
Genes are sequences of DNA on chromosomes that encode a functional product. An allele is a form of a gene present at a particular location (locus) on the chromosome. Since human cells are diploid, each locus is present as two alleles, which may or may not be identical to each other.
Mitosis is the process whereby a cell divides into two daughter cells such that each daughter cell contains the same number and kind of chromosomes as the parent cell. Human cells contain 46 chromosomes; during the phases of mitosis, the chromosomes are copied and the cell then divides, sending 46 chromosomes to each daughter cell.
Meiosis is the process whereby a cell produces four daughter cells, each with a haploid number of chromosomes (one-half the number possessed by the parent cell). Meiosis is utilized for the production of haploid germ cells (sperm and eggs).
Mendelian inheritance patterns include autosomal dominant, autosomal recessive, X-linked dominant, and X-linked recessive. An autosomal dominant mutation only needs to be present in one copy of a gene in the genome (it is dominant to the normal allele on the other chromosome) for the effects of the mutation to be evident. It is inherited 50% of the time from the parent with the disease. An autosomal recessive mutation means that for the disease to be manifest both alleles must contain a mutation, and each parent contributes one mutated allele to the fetus. The probability of inheriting an autosomal recessive disease is 25%, as there is a 1 in 2 chance that each parent will pass on the allele, and both events must occur for the child to inherit two mutant alleles (one-half times one-half is one-quarter). X-linked disorders are due to mutations in genes residing on the X chromosome. X-linked recessive disorders are inherited by males from carrier or affected females, and since males only contain one X chromosome they express the disease. X-linked dominant disorders are expressed by both males and females.
Failure of the chromosomes to sort appropriately during meiosis can lead to nondisjunction events, which allow germ cells with an abnormal number of individual chromosomes to be created (aneuploidy), and often leads to spontaneous abortion or disease. Gene dosage effects are important as over expression of genes can be detrimental, as can reduced expression of genes (due to fewer chromosomes carrying the allele). Also, during meiosis, chromosome structure can be altered, leading to inversions, duplications, insertions, isochromosome formation, deletions, and translocations.
The Hardy–Weinberg equilibrium allows one to determine estimates of allele frequencies in a general population, which can generate the frequency of heterozygotes in a population, as well as the frequency of those affected by a disease. It is best applied to autosomal and X-linked recessive disorders.
Multifactorial diseases (such as schizophrenia) involve significant interactions between multiple genes and environmental factors.
DNA structural abnormalities can also lead to disease. An example of such an abnormality is triplet nucleotide repeat expansion within or near certain genes. If the DNA expansion exceeds a certain size, disease can result; such diseases are inherited in an autosomal dominant fashion. Anticipation refers to an earlier onset of disease and more severe symptoms in members of later generations, which correlates with an increase in the number of triplet nucleotide repeats in successive generations.
Tumor suppressor genes (genes whose normal function is to block uncontrolled cellular proliferation), if analyzed through pedigrees, most often display an autosomal dominant pattern of inheritance, yet the molecular mechanism is recessive. The loss of a functional allele is known as loss of heterozygosity and occurs through a variety of mechanisms.
THE WAITING ROOM 
Carrie S., Will S.’s 19-year-old sister, is considering marriage. Her growth and development have been normal, and she is free of symptoms of sickle-cell anemia. Because a younger sister, Amanda, was tested and found to have sickle-cell trait (expressing one normal β-globin gene, and one sickle β-globin gene), and because of Will S.’s repeated sickle-cell crises, Carrie S. wants to know whether she also has sickle-cell trait (see Chapters 6 and 7 for Will S.’s history). A hemoglobin electrophoresis is performed that shows the composition of her hemoglobin to be 58% HbA, 39% HbS, 1% HbF, and 2% HbA2, a pattern consistent with the presence of sickle-cell trait. The hematologist who saw her in the clinic on her first visit is studying the genetic mutations of sickle-cell trait and asks Carrie S. for permission to draw additional blood for more sophisticated analysis of the genetic disturbance that causes her to produce HbS. Carrie S. informed her fiancé that she has sickle-cell trait and that she wants to delay their marriage until he is tested.
Martha W. is one of five siblings who express symptoms of a very rare mitochondrial disorder MERRF (myoclonic epilepsy with ragged red fibers). Mitochondrial diseases occur due to mutations in the mitochondrial genome and display maternal inheritance. Because of heteroplasmy, the symptoms of MERRF vary widely among affected individuals, even in the same family. Martha W. has the mildest case of her siblings experiencing some muscle weakness and muscle twitching. Unfortunately, her other siblings have more severe symptoms and two of her siblings died in their teens from the disease. Martha W.’s mother also has a mild case of the disorder.
Martha W. has just recently married and would like children of her own, but knowing that as a woman with a mitochondrial disorder she will transmit the disease to all her children, she would like to do her best to prevent her children from inheriting her disease. A search on the internet leads her to a foreign clinic for a consultation with a doctor who claims to be able to help women with mitochondrial disorders have children without the disease.
I. Mendelian Inheritance Patterns
Humans are diploid organisms, meaning that each somatic cell contains two copies of each chromosome, one from each parent. The cells contain 46 chromosomes (two copies of each autosomal chromosome, numbered from 1 through 22, and two sex chromosomes, which can be either XX or XY). As females are XX, the egg contains one copy each of chromosomes 1 through 22, and one X chromosome. Sperm contain one copy each of chromosomes 1 through 22, and either one X or one Y chromosome. Fertilization of an egg by a sperm will lead to a zygote with 46 chromosomes, which then develops into a fetus and then an infant.
Ploidy refers to the copy number of the chromosome complement in multiples of 23 chromosomes in human cells. Monoploid refers to a cell with just one copy of all chromosomes; diploid refers to two copies, and triploid (69 chromosomes) refers to cells with three copies of all chromosomes. Only diploid cells are viable. Aneuploidy refers to an abnormal number of individual chromosomes rather than a multiple of 23. Loss of a chromosome is considered aneuploidy (Turner syndrome, which is monosomy X [45 XO], is considered an aneuploid disorder), as is the gain of a chromosome (Down syndrome, trisomy 21 [47, XX, +21; 47, XY, +21], is an aneuploid disorder).
Cellular division involves replication of the DNA within the nucleus of a cell and successful transfer of one intact copy of the duplicated genome to the daughter cells. This process is known as mitosis (see Chapter 10) and consists of a number of different stages during which the chromosomes are duplicated, condensed, and copies are sent to the appropriate daughter cells (Fig. 18.1). The generation of gametes (germ cells) requires the process of meiosis, in which the chromosomes are duplicated (making the cell tetraploid) and then a first meiotic division occurs in which two sister chromatids are sent into daughter cells (in mitosis the sister chromatids are split and go into different daughter cells). The second meiotic division splits the sister chromatids such that each germ cell gets a haploid number of chromosomes. An important principle is that of independent assortment, which states that during meiosis each chromosome in a pair is randomly sorted into a daughter cell. There is no linkage between chromosomes when they segregate during meiosis, thereby providing up to 2 to the 22nd power in combinations of chromosomes in the resulting gametes (excluding the sex chromosomes).
FIGURE 18.1 A. The process of mitosis. For simplicity, only four chromosomes are shown. Note that when the chromosomes separate to be passed to daughter cells (from 4N to 2N ploidy), the sister chromatids are separated during cell division to reach the diploid state. B. The steps of meiosis, in which a 2N cell increased ploidy to 4N, then decreases, via two meiotic divisions, back to 2N and finally to an N content of DNA. Note that in meiosis I, the 2N content of DNA consists of sister chromatids (basically identical chromosomes) that are separated in the second meiotic division to form cells with an N content of DNA. C. Crossing over during meiosis. Two pairs of sister chromatids can exchange genetic information before the first meiotic division. (Reprinted with permission from Gelehrter TD, Collins FS, Ginsburg D. Principles of Medical Genetics. 2nd ed. Williams & Wilkins; 1998. Figures 2.11 and 2.12.)
An important feature of meiosis 1 is that before metaphase 1, but after DNA replication, crossover of genetic information occurs between paired homologous chromosomes. Such crossover events increase genetic diversity by altering the combination of genes on homologous chromosomes, which will be separated into two different cells during the second meiotic division. There are approximately 3 to 5 crossover events per chromosome during meiosis.
Chromosomes can be visualized by karyotype, which displays all of the chromosomes in a cell, obtained through a metaphase spread (Fig. 18.2). Alterations in karyotype (gain or loss of chromosomes, large insertions or deletions, and translocations), can be easily observed through this technique. Tumor cells often exhibit genomic instability and can have quite complicated karyotypes.
FIGURE 18.2 An example of a male karyotype (note the presence of an X and a Y chromosome). (Reprinted with permission from Lieberman MA, Ricer R. BRS Biochemistry, Molecular Biology & Genetics. 7th ed. Wolters Kluwer; 2020. Figure 10.1.)
II. Genes
Genes, the basic unit of heredity, reside at specific locations, known as loci (plural) or locus (singular), on a particular chromosome. The form of a gene at a given locus is an allele. The homozygous state refers to the two alleles being identical; the heterozygous state refers to the two alleles having a different nucleotide sequence, which may be caused by mutations. A phenotype refers to the observable traits of the individual, produced by the interaction of the individual’s genes and the environment. Genotype refers to the genetic composition of the individual.
The ability to inherit a trait from a parent depends on two variables: a genetic component and an environmental component. Together they define heritability. A genetic component of 100% indicates no influence of the environment on the inheritance of the phenotype of the individual. A genetic component of 10% would indicate the major determinant of the phenotype is environmental rather than genetic.
Autosomal alleles are present as pairs within cells. If the alleles are different what determines which allele is expressed? This is determined by whether one allele is dominant, or recessive, to the other, or if they are codominant and equally expressed. A dominant trait is one that manifests itself even when an allele is in a heterozygous state (one example of this is a protein composed of subunits—having a mix of normal and altered subunits may render the entire complex inactive). Codominant traits are when both alleles in a heterozygous pair are expressed (one example is the blood group antigens, such that a person can express both an A antigen and a B antigen—type AB blood). A recessive trait is one that manifests only when the gene is in a homozygous state (an example is sickle-cell disease, where the disease is expressed when both alleles contain the same mutation, which produces an altered β subunit of hemoglobin). Recessive X-linked traits occur when a mutant allele is located on the X chromosome and will be expressed in males (since males only have one X chromosome), but not usually in females. Females with one mutant allele on an X chromosome are carriers of the disorder and do not express the disease if the nonmutated allele on the other X chromosome is dominant to the mutated allele. X-linked traits also can be dominant; if dominant then both males and females who inherit the mutant allele will express the trait.
Not all individuals who inherit a mutant allele will necessarily express the phenotype of the disease. The term penetrance refers to the probability an individual will express a phenotype when inheriting a particular mutant allele. A penetrance of 100% means that everyone who inherits the mutant allele will express the disease. Penetrance can be <100% due to, in part, the nature of the mutation in the allele, epigenetic differences between individuals, or differences in modifier genes between individuals. Breast cancer, due to mutations in BRCA1 or BRCA2, displays incomplete penetrance. Variable expressivity refers to the severity of the expressed phenotype caused by a mutant allele. Even if the penetrance of a particular mutant allele is 100%, the allele may lead to different phenotypes (variable expressivity) in members of the same family who inherit the allele. Variable expressivity is often caused by environmental influences and modifier genes that affect the phenotype. Marfan’s syndrome and osteogenesis imperfecta are diseases that display variable expressivity.
III. Mutations
Alterations in a DNA sequence of an allele can give rise to a nonfunctional or unregulated gene product (see Chapter 15). Mutations can be classified in a variety of ways, as indicated in Table 18.1. All newborn infants are tested for genetic diseases, such as phenylketonuria (PKU), although the diseases tested for by newborn screening vary by state. Many genetic disorders require early treatment to prevent serious and irreversible consequences to the infant. The U.S. federal government has a recommended list of 35 disorders to be tested, but each state makes its own determination.
TYPE OF MUTATION | DESCRIPTION | EXAMPLE |
Point mutations | A change in one base of the DNA | Sickle-cell disease |
Deletions | A loss of bases in the DNA | Cystic fibrosis (ΔF508); infantile Tay–Sachs disease |
Insertions | New DNA sequence added into the existing DNA | A form of Menkes disease (a disorder of copper metabolism) |
Loss of a chromosome | Creates an aneuploid state | Turner syndrome |
Extra copy of a chromosome | Trisomy for an autosomal chromosome leads to disease | Down syndrome |
Trinucleotide repeats | Expansion of specific trinucleotide sequences in a gene can give rise to a disease | Huntington disease |
Epigenetic | No alterations in base sequence but chemical modifications to the DNA and histones do occur (gain or loss of methylation and acetylation patterns, for example) | Angelman and Prader–Willi syndromes |
IV. Inheritance Patterns
Mutations can be inherited, in Mendelian fashion, via autosomal dominant, autosomal recessive, and X-linked mechanisms. Non-Mendelian inheritance includes mitochondrial disorders, due to mutations in the mitochondrial genome. Each will be described below, along with examples of diseases transmitted via that mechanism.
A. Autosomal Dominant Inheritance
A sample pedigree of autosomal dominant inheritance is shown in Figure 18.3A along with the common symbols used in pedigrees (Fig. 18.3B). An affected individual most often has an affected parent (unless the affected individual is expressing a new mutation). The affected individuals are heterozygotes, as homozygosity for these traits is statistically very unlikely, and may be lethal in utero. An affected heterozygous parent has a 50% chance of passing the affected allele to their offspring. Transmission of the trait is sex-independent, and both sexes can express the disease.
FIGURE 18.3 A. An autosomal dominant pedigree. The large “A” is the dominant mutant allele, and the small “a” is the wild type allele. The darkened boxes indicate the individuals with the disease. B. Common symbols used in pedigree analysis. (Reprinted with permission from Lieberman MA, Ricer R. BRS Biochemistry, Molecular Biology & Genetics. 7th ed. Wolters Kluwer; 2020. Figures 10.2 and 10.3.)
A Punnett square analysis will aid in calculating the probabilities of passing the altered allele to the children (Fig. 18.4). For an autosomal dominant pattern of inheritance 50% of the children will be affected, whereas 50% will not acquire the mutated gene (thus, there is a one in two chance of inheriting the mutated allele).
FIGURE 18.4 A Punnett square analysis of an autosomal dominant disorder. The disease gene is indicated by the uppercase “A.” Note that 50% of the offspring will inherit the disease, as any individual with at least one copy of the “A” allele will express the disease.
B. Autosomal Recessive Inheritance
A sample pedigree of autosomal recessive inheritance is shown in Figure 18.5A. Indications of autosomal recessive inheritance include both sexes being affected equally when inheriting the mutant alleles, that the transmission of the trait must occur from both parents, and that generations may be skipped in the expression of the disease if all offspring are heterozygotes. Figure 18.5B displays a Punnett square analysis of autosomal recessive inheritance. Note that one in four children (25%) will be affected, and two in four children (50%) will be a carrier of the disease.
FIGURE 18.5 A. An autosomal recessive inheritance pedigree. The lowercase “a” reflects the disease allele; a person with the genotype aa will express the disease, whereas the genotype Aa designates a carrier of the disease. B. A Punnett square analysis of autosomal recessive inheritance. Note that one in four of the offspring will inherit both mutated alleles, and express the disease. (Reprinted with permission from Lieberman MA, Ricer R. BRS Biochemistry, Molecular Biology & Genetics. 7th ed. Wolters Kluwer; 2020. Figures 10.5 and 10.6.)
C. X-linked Inheritance
X-linked inheritance refers to inheritance of mutant alleles on the X chromosome. Males are hemizygous for genes on the X chromosome, as males only have one copy of the X chromosome, while females have two copies of the X chromosome. For X-linked recessive disorders, there is no male-to-male transmission in a pedigree (Fig. 18.6A), and females are usually asymptomatic (for an exception see the Lyon hypothesis, below). Sons and daughters each have a 50% chance of inheriting the mutant allele from their mothers, but the sons will express the disease while the daughters will be carriers of the disease. The Punnett square analysis of X-linked recessive disorders is shown in Figure 18.6B.
FIGURE 18.6 A. A pedigree demonstrating X-linked recessive inheritance. Females with one defective allele are carriers of the disease. B. A Punnett square analysis of X-linked recessive allele transmission. In this case, the lowercase “x” refers to the X chromosome carrying the mutant allele. Partially shaded symbols indicate a carrier of the mutation, whereas fully shaded symbols indicate an individual who expresses the disease. (Reprinted with permission from Lieberman MA, Ricer R. BRS Biochemistry, Molecular Biology & Genetics. 7th ed. Wolters Kluwer; 2020. Figures 10.7 and 10.8.)
1. Gene Dosage and the Lyon Hypothesis
The expression of genes on an extra chromosome copy (trisomy), or loss of expression of genes on a chromosome (monosomy, due to a chromosomal deletion) is detrimental for human development. All monosomies of an entire autosomal chromosome (chromosomes 1 to 22) are embryonic lethal, whereas trisomic conditions of chromosomes 13, 18, and 21 are tolerated to a limited extent, but trisomies of other chromosomes do not result in viable offspring. Monosomic X chromosomes give rise to viable female offspring, but they do exhibit a phenotype.
The X chromosome is about five times larger than the Y chromosome, and if females expressed all of the genes on both X chromosomes, they would express many genes much more highly than males. To compensate for this difference in gene expression from the sex chromosomes, and to keep gene dosage equal between the sexes, X-chromosome inactivation occurs when there is more than one X chromosome in a somatic cell. For females with 2 X chromosomes, one X chromosome in each cell is inactivated and condensed. The resulting chromosome copy is known as a Barr body. Inactivation is random as to the origin (maternal or paternal) of the chromosome (Fig. 18.7). While the Barr body is highly condensed a small region of the chromosome is transcriptionally active, to balance the level of transcription from a male’s Y chromosome.
FIGURE 18.7 The Lyon hypothesis. The dark bar represents the inactivated chromosomes. The Lyon hypothesis explains how equal numbers of active genes are maintained in males and females. Inactivation at the 16-cell stage is random. Once an X chromosome is inactivated in a cell, all subsequent daughter cells have the same pattern of X-inactivation. In the zygote, both maternal (Xm) and paternal (Xp) X chromosomes are active. (Reprinted with permission from Lieberman MA, Ricer R. BRS Biochemistry, Molecular Biology & Genetics. 7th ed. Wolters Kluwer; 2020. Figure 10.9.)
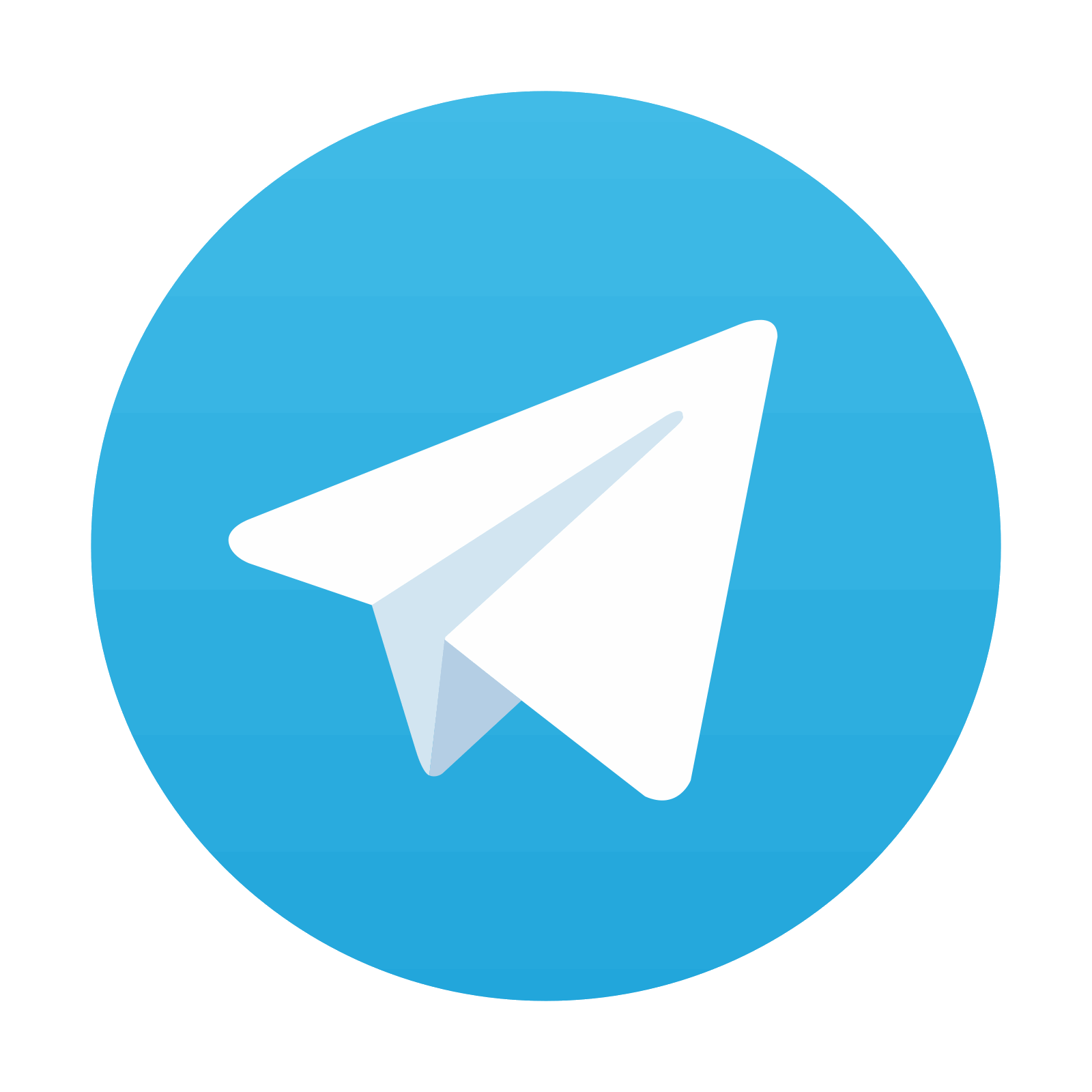
Stay updated, free articles. Join our Telegram channel
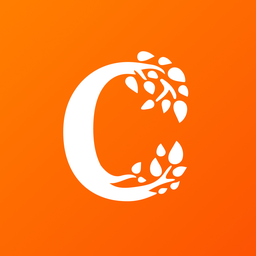
Full access? Get Clinical Tree
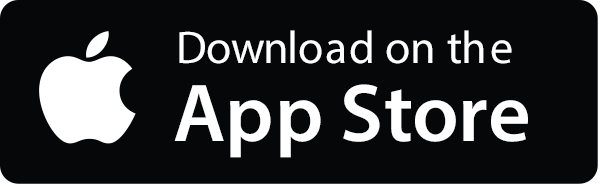
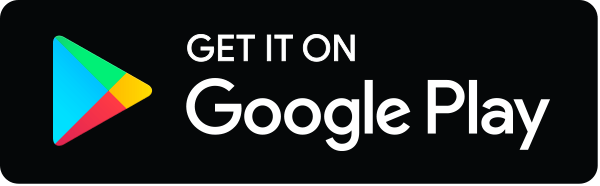