Nucleotides in DNA (deoxyribonucleic acid) and RNA (ribonucleic acid). Nucleotides are the monomeric units of the nucleic acids, DNA (deoxyribonucleic acid) and RNA (ribonucleic acid). Each nucleotide consists of a heterocyclic nitrogenous base, a sugar, and phosphate. DNA contains the purine bases adenine (A) and guanine (G) and the pyrimidine bases cytosine (C) and thymine (T). RNA contains A, G, and C, but it has uracil (U) instead of thymine. In DNA, the sugar is deoxyribose, whereas in RNA it is ribose.
Polynucleotides such as DNA and RNA are linear sequences of nucleotides linked by 3′- to 5′-phosphodiester bonds between the sugars (Fig. 11.1). The bases of the nucleotides can interact with other bases or with proteins.
FIGURE 11.1 Structure of a polynucleotide. The 5′-carbon of the top sugar and the 3′-carbon of the bottom sugar are indicated. Because the sugar is ribose, this is an example of RNA.
DNA Structure. Genetic information is encoded by the sequence of different nucleotide bases in DNA. DNA is double stranded; it contains two antiparallel polynucleotide strands. The two strands are joined by hydrogen bonding between their bases to form base pairs (bp). Adenine pairs with thymine, and guanine pairs with cytosine. The two DNA strands run in opposite directions. One strand runs 5′ to 3′, and the other strand runs 3′ to 5′. The two DNA strands wind around each other, forming a double helix.
Transcription of a gene generates a single-stranded RNA that is identical in nucleotide sequence to one of the strands of the duplex DNA (except for U in RNA where T is in DNA). The three major types of RNA are messenger RNA (mRNA), ribosomal RNA (rRNA), and transfer RNA (tRNA).
RNA Structures. mRNAs contain the nucleotide sequence that is converted into the amino acid sequence of a protein in the process of translation. Eukaryotic mRNA has a structure known as a cap at the 5′-end, a sequence of adenine nucleotides (a poly[A] tail) at the 3′-end, and a coding region in between containing codons that dictate the sequence of amino acids in a protein or relay a signal. Each codon in the genetic code is a different sequence of three nucleotides.
rRNAs and tRNAs are part of the apparatus for protein synthesis, but they do not encode proteins. rRNA has extensive internal base pairing and complexes with proteins to form ribonucleoprotein particles called ribosomes. The ribosomes bind mRNA and tRNAs during translation. Each tRNA binds and activates a specific amino acid for insertion into the polypeptide chain and therefore has a somewhat different nucleotide sequence than other tRNAs. A unique trinucleotide sequence on each tRNA called an anticodon binds to a complementary codon on the mRNA, thereby ensuring insertion of the correct amino acid. In spite of their differences, all tRNAs contain a number of unusual nucleotides and assume a similar cloverleaf structure.
THE WAITING ROOM 
Isabel S. is a 26-year-old who suffers from opioid use disorder and uses intravenous (IV) opioids. She presented to the emergency department with a painful, swollen left arm and was found to have an abscess on her arm where she injects. She reported that a few months ago, she had a 3-week course of a flulike syndrome with fever, malaise, and muscle aches. She was offered an HIV (human immunodeficiency virus) test, which was positive and a multidrug regimen was initiated.
Clark T. had a screening colonoscopy at age 50 where they removed several intestinal polyps, whose pathology was consistent with adenomas. He did not return for a 3-year colonoscopic examination as instructed for surveillance. At age 59, he reappeared, complaining of maroon-colored stools, an indication of intestinal bleeding. The source of the blood loss was an adenocarcinoma growing from a colonic polyp of the large intestine. A computed tomography (CT) scan of the abdomen and pelvis showed the tumor likely invading the gut wall with enlarged pericolic lymph nodes and several small masses in the liver, which confirmed metastatic cancer. Following resection of the tumor in both the colon and liver, the oncologist began treatment with 5-fluorouracil (5-FU) combined with other chemotherapeutic agents.
Paul T. complains to his physician of a 3-day history of worsening fever and cough. His cough produces thick yellow-brown sputum. He has crackles on pulmonary examination at the right base of his lungs. A chest X-ray is ordered and demonstrates an infiltrate in the right base consistent with pneumonia, and he is empirically started on azithromycin for community-acquired pneumonia. His physician was able to obtain a sputum sample from Paul T., which was sent for stain and culture. The stain of his sputum shows many gram-positive diplococci. A sputum culture is initiated to determine whether he has been infected with Streptococcus pneumonia.
I. DNA Structure
A. Location of DNA
DNA and RNA serve as the genetic material for prokaryotic and eukaryotic cells, viruses, and plasmids, each of which stores it in a different arrangement or location. In prokaryotes, DNA is not separated from the rest of the cellular contents. In eukaryotes, however, DNA is located in the nucleus, where it is separated from the rest of the cell by the nuclear envelope (see Fig. 10.9) and in the mitochondria. Eukaryotic nuclear DNA is bound to proteins, forming a complex called chromatin. During interphase (when cells are not dividing), some of the chromatin are diffuse (euchromatin) and some are dense (heterochromatin), but no distinct structures can be observed. However, before mitosis (when cells divide), the DNA is replicated, resulting in two identical chromosomes called sister chromatids. During metaphase (a period in mitosis), these condense into discrete, visible chromosomes.
DNA is a double-stranded molecule that forms bp, via hydrogen bonding, between strands (see section I.C). The bp designation is often used to indicate the size of a DNA molecule. For example, in a stretch of DNA 200 bp long, both strands are included, with 200 bases in each strand, for a total of 400 bases.
Less than 0.1% of the total DNA in a cell is present in mitochondria. The genetic information in a mitochondrion is encoded in <20,000 bp of DNA; the information in a human haploid nucleus (i.e., an egg or a sperm cell) is encoded in about 3 × 109 (3 billion) bp. The DNA and protein-synthesizing systems in mitochondria more closely resemble the systems in bacteria, which do not have membrane-enclosed organelles, than those in the eukaryotic nucleus and cytoplasm. It has been suggested that mitochondria were derived from ancient bacterial invaders of primordial eukaryotic cells.
Plasmids are small, circular DNA molecules that can enter bacteria and replicate autonomously, that is, outside the host genome. In contrast to viruses, plasmids are not infectious; they do not convert their host cells into factories devoted to plasmid production. Plasmids do, however, often carry genes, some of which confer resistance to antibiotics. Genetic engineers utilize plasmids as tools for transfer of foreign genes into bacteria because segments of DNA can readily be incorporated into plasmids.
B. Determination of the Structure of DNA
In 1869, Friedrich Miescher first isolated DNA, obtaining it from pus scraped from surgical bandages. Initially, scientists speculated that DNA was a cellular storage form for inorganic phosphate, an important but unexciting function that did not spark widespread interest in determining its structure. In fact, the details of DNA structure were not fully determined until 1953, almost 90 years after it had first been isolated, but only 9 years after it had been identified as the genetic material.
Early in the 20th century, the bases of DNA were identified as the purines adenine (A) and guanine (G), and the pyrimidines cytosine (C) and thymine (T) (Fig. 11.2). The sugar was found to be deoxyribose, a derivative of ribose, lacking a hydroxyl group on carbon 2 (see Fig. 11.2).
FIGURE 11.2 A. Purine and pyrimidine bases in DNA. B. Deoxyribose and ribose, the sugars of DNA and RNA. The carbon atoms are numbered from 1 to 5. When the sugar is attached to a base, the carbon atoms are numbered from 1′ to 5′ to distinguish it from the base. In deoxyribose the X = H; in ribose the X = OH.
Nucleotides, composed of a base, a sugar, and phosphate, were found to be the monomeric units of the nucleic acids (Table 11.1). In nucleosides, the nitrogenous base is linked by an N-glycosidic bond to the anomeric carbon of the sugar, either ribose or deoxyribose. The atoms in the sugar are numbered using the prime symbol (′) to distinguish them from the numbering of the atoms in the nitrogenous base. A nucleotide is a nucleoside with an inorganic phosphate attached to a 5′-hydroxyl group of the sugar in ester linkage (Fig. 11.3). The names and abbreviations of nucleotides specify the base, the sugar, and the number of phosphates attached (MP, monophosphate; DP, diphosphate; TP, triphosphate). In deoxynucleotides, the prefix “d” precedes the abbreviation. For example, GDP is guanosine diphosphate (the base guanine attached to a ribose that has two phosphate groups) and dATP is deoxyadenosine triphosphate (the base adenine attached to deoxyribose with three phosphate groups).
TABLE 11.1 Names of Bases and Their Corresponding Nucleosidesa
BASE | NUCLEOSIDE |
Adenine (A) | Adenosine |
Guanine (G) | Guanosine |
Cytosine (C) | Cytidine |
Thymine (T) | Thymidine |
Uracil (U) | Uridine |
Hypoxanthine (I) | Inosineb |
aIf the sugar is deoxyribose rather than ribose, the nucleoside has “deoxy” as a prefix (e.g., deoxyadenosine). Nucleotides are given the name of the nucleoside plus mono-, di-, or triphosphate (e.g., adenosine triphosphate or deoxyadenosine triphosphate).
bThe base hypoxanthine is not found in DNA but is produced during the degradation of the purine bases. It is found in certain tRNA molecules. Its nucleoside, inosine, is produced during the synthesis of the purine nucleotides (see Chapter 41).
FIGURE 11.3 Nucleoside and nucleotide structures displayed with ribose as the sugar. The corresponding deoxyribonucleotides are abbreviated dNMP, dNDP, and dNTP. N = any base (A, G, C, U, or T). The hydrogen atoms (H) have been removed from the figure to improve clarity.
In 1944, after Oswald Avery’s experiments establishing DNA as the genetic material were published, interest in determining the structure of DNA intensified. Digestion with enzymes of known specificity proved that inorganic phosphate joined the nucleotide monomers, forming a phosphodiester bond between the 3′-carbon of one sugar and the 5′-carbon of the next sugar along the polynucleotide chain (Fig. 11.4). Another key to DNA structure was provided by Erwin Chargaff. He analyzed the base composition of DNA from various sources and concluded that on a molar basis the amount of adenine was always equal to the amount of thymine, and the amount of guanine was equal to the amount of cytosine.
FIGURE 11.4 A segment of a polynucleotide chain of DNA. The dashes at the 5′- and 3′-ends indicate that the molecule contains more nucleotides than are shown. The hydrogen atoms (H) have been omitted from the sugar structures to increase the clarity of the figure.
During this era, James Watson and Francis Crick joined forces and, using the X-ray diffraction data of Maurice Wilkins and Rosalind Franklin, incorporated the available information into a model for DNA structure. In 1953, they published a brief paper (approximately 900 words), describing DNA as a double helix consisting of two polynucleotide strands joined by pairing between the bases (adenine with thymine and guanine with cytosine). The model of base pairing they proposed and the implications of the model for understanding DNA replication formed the basis of modern molecular biology.
C. Concept of Base Pairing
As proposed by Watson and Crick, each DNA molecule consists of two polynucleotide chains joined by hydrogen bonds between the bases. In each bp, a purine on one strand forms hydrogen bonds with a pyrimidine on the other strand. In one type of bp, adenine on one strand pairs with thymine on the other strand (Fig. 11.5). This bp is stabilized by two hydrogen bonds. The other bp, formed between guanine and cytosine, is stabilized by three hydrogen bonds. As a consequence of base pairing, the two strands of DNA are complementary; that is, adenine on one strand corresponds to thymine on the other strand, and guanine corresponds to cytosine.
FIGURE 11.5 Base pairs of DNA. Note that the purine bases are “flipped over” from the positions in which they are usually shown (see Fig. 11.4). The bases must be in this orientation to form base pairs. The dotted lines indicate hydrogen bonds between the bases. Although the hydrogen bonds participate in holding the bases and thus the two DNA strands together, they are weaker than covalent bonds and allow the DNA strands to separate during replication and transcription.
The concept of base pairing proved to be essential for determining the mechanism of DNA replication (in which the copies of DNA produced are distributed to daughter cells) and the mechanisms of transcription and translation (in which mRNA is produced from genes and used to direct the process of protein synthesis). As Watson and Crick implied in their article, base pairing allows one strand of DNA to serve as a template for the synthesis of the other strand (Fig. 11.6). Base pairing also allows a strand of DNA to serve as a template for the synthesis of a complementary strand of RNA.
FIGURE 11.6 DNA strands serve as templates. During replication, the strands of the helix separate in a localized region. Each parental strand serves as a template for the synthesis of a new DNA strand.
D. DNA Strands Are Antiparallel
As concluded by Watson and Crick, the two complementary strands of DNA run in opposite directions (antiparallel). On one strand, the 5′-carbon of the sugar is above the 3′-carbon (Fig. 11.7). This strand is said to run in a 5′-to-3′ direction. On the other strand, the 3′-carbon is above the 5′-carbon. This strand is said to run in a 3′-to-5′ direction. Thus, the strands are antiparallel (i.e., they run in opposite directions). This concept of directionality of nucleic acid strands is essential for understanding the mechanisms of replication and transcription.
FIGURE 11.7 Antiparallel strands of DNA. For the strand on the left, the 5′-carbon of each sugar is above the 3′-carbon, so the 5′-to-3′ direction is from top to bottom. For the strand on the right, the 5′-carbon of each sugar is below the 3′-carbon, so the 5′-to-3′ direction is from bottom to top.
E. The Double Helix
Because each bp contains a purine bonded to a pyrimidine, the strands are equidistant from each other throughout. If two strands that are equidistant from each other are twisted at the top and the bottom, they form a double helix (Fig. 11.8). In the double helix of DNA, the bp that join the two strands are stacked like a spiral staircase along the central axis of the molecule. The electrons of the adjacent bp interact, generating hydrophobic stacking forces that, in addition to the hydrogen bonding of the bp, stabilize the helix.
FIGURE 11.8 A. Two DNA strands twist to form a double helix. The distance between the two phosphodiester backbones is about 11 Å. The hydrogen-bonded base pairs, shown bonded by dotted lines, create stacking forces with adjacent base pairs. Each phosphate group contains one negatively charged oxygen atom that provides the phosphodiester backbone with a negative charge. Because of the twisting of the helix, grooves are formed along the surface, the larger one being the major groove and the smaller one the minor groove. B. If you look up through the bottom of a helix along the central axis and the helix spirals away from you in a clockwise direction (toward the arrowhead in the drawing), it is a right-handed helix. If it spirals away from you in a counterclockwise direction, it is a left-handed helix.
The phosphate groups of the sugar–phosphate backbones are outside the helix (see Fig. 11.8). Each phosphate has two oxygen atoms forming the phosphodiester bonds that link adjacent sugars. However, the third –OH group on the phosphate is free and dissociates a hydrogen ion at physiologic pH. Therefore, each DNA helix has negative charges coating its surface, which facilitate the binding of specific proteins.
The helix contains grooves of alternating size, known as the major and minor grooves (see Fig. 11.8). The bases in these grooves are exposed and therefore can interact with proteins or other molecules.
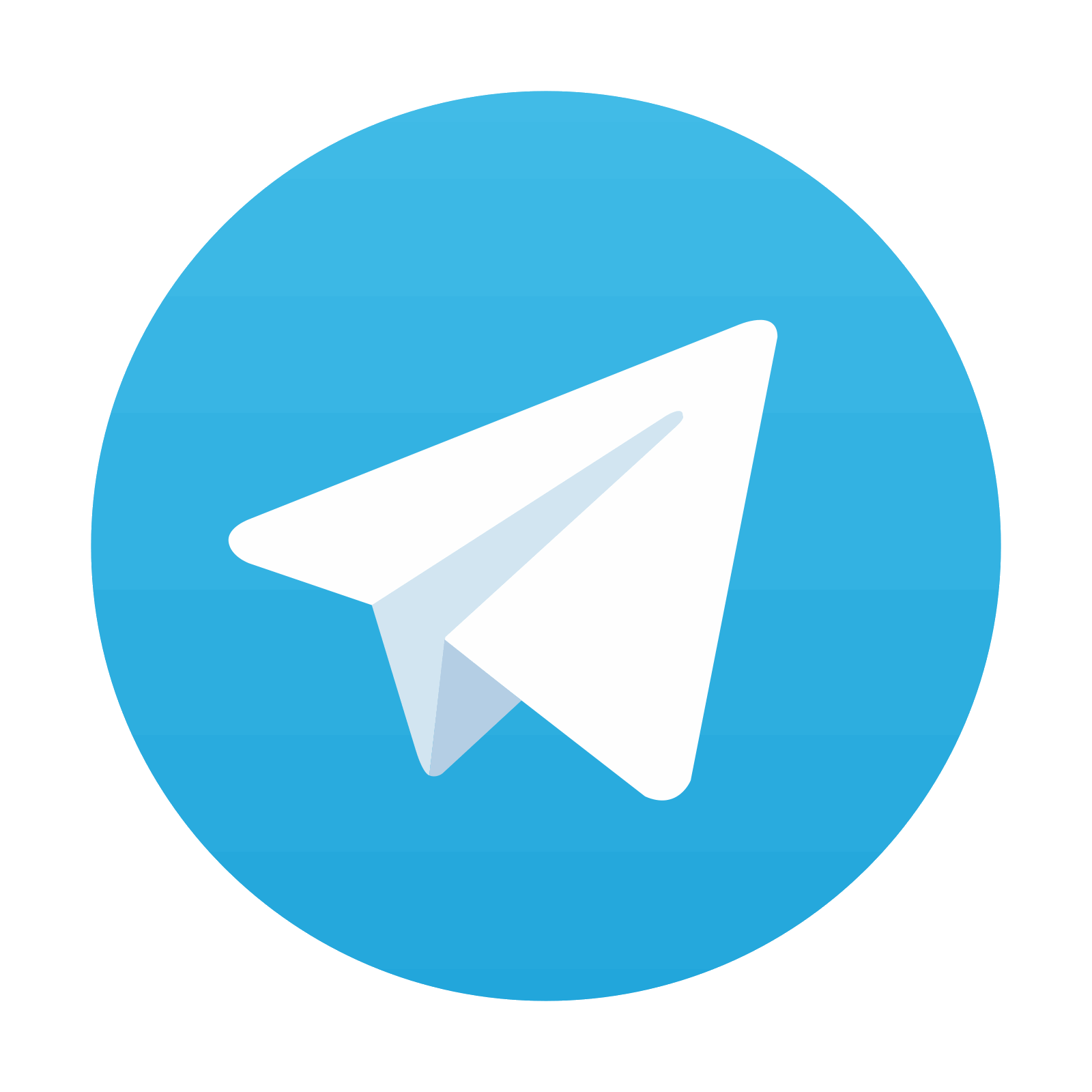
Stay updated, free articles. Join our Telegram channel
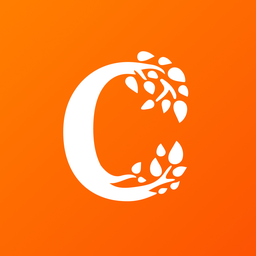
Full access? Get Clinical Tree
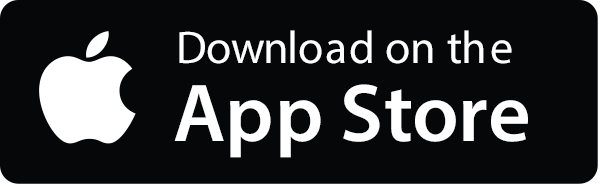
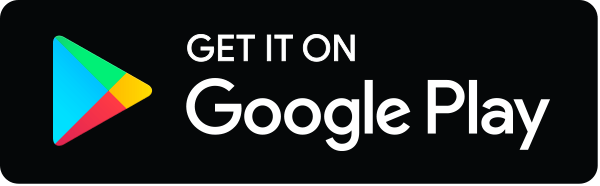