6 Monica Berry1 and Anthony Corfield2 1Department of Physics University of Bristol UK 2School of Clinical Sciences University of Bristol UK Mucosal surfaces, wet epithelia covered by a protective and dynamic gel blanket, are found at the border of self and nonself wherever transparency, chemical sensing, gas exchange and absorption take place, and where lubrication is essential for the physiological function of the organ (Figure 6.1). Figure 6.1 Mucin gels in the human body. Mucin gels are found at all boundaries between self and environment that are not covered by skin. Thus, the surface of the eyes, the respiratory tract from the nasal passage to bronchioles (but not alveoli), the gastrointestinal tract from mouth to anus, and the reproductive tract, are all protected and lubricated by mucus gels with properties specific to their location. Lumens of airways, gastrointestinal and genitourinary tracts, as well as the surface of the eyes are targets for drug delivery where mucoadhesion promises to improve the effect of medication. In addition to tissues that can be directly reached by topical medication, that is the external eye, nose, oral cavity, airways and upper gastrointestinal tract, mucosal surfaces can serve as portals to the systemic circulation (e.g. the nasal mucosa) or to other tissues; for example, through a trans-conjunctival route to the retina, colon via the rectum or upper reproductive tract through the vaginal mucosa. As well as widening the scope of topical therapy, mucosal gels can work against the therapeutic agent because of their continuous renewal through secretion and degradation. The latter is effected by glycosidases and proteases that might also accept other polymers as substrates, the presence of beating cilia that eliminate particulates in a size-dependent fashion, as well as by nonspecifically adhering to, wrapping around and removing particulate matter from the gel. Interaction or interweaving of polymers that coat a nanoparticle, for example, can alter its penetration towards the epithelial target, in ways that range from lubricating its way to the total immobillisation of the nanoparticle. Changes in pH and ionic strength may help or hinder either directly or through effects on the pore size of the mucus. This chapter presents the architectural features underpinning the physiological roles of mucins, a large family of heavily O-glycosylated macromolecules that are the main functional component of mucosal gels and underpin mucoadhesion. However, it should be kept in mind that mucins alone cannot duplicate the characteristics of the gel [1]. As is shown later, some structural features of mucins can be found in unrelated molecules that fulfil different functions, for example cysteine domains in a cartilage protein or the Tunicate protein, oikosin [2]; mucin domains are found in a number of proteins, for example fractalkine and P-selectin – involved in adhesion, or in TIM1 and TIM3 – molecules that affect immune function [3]. These molecules might become therapy targets themselves, perhaps using chemistry related to that promoting mucoadhesion. The mucin gene family (the MUC genes) codes for 21 or more proteins that are expressed on a tissue specific basis. Three main groups have been identified and these are shown in Table 6.1. Table 6.1 The mucin gene family; chromosomal location and tandem repeat size The first group is the secreted, gel forming mucins. The second group is represented by MUC7, a secreted, nongel-forming mucin, MUC8, which is still poorly characterised, and MUC9. The third and most abundant group is the membrane-associated mucins [4–7]. The mucins are very large, multipeptide domain, linear polymers and have been categorised on the basis of their peptide domain organisation. The unique molecular structure enables the formation of networks for the secreted forms and a contribution to the glycocalyx at the cell surface by membrane-spanning mucins. A feature of this organisation is homo-oligomerisation. None of the MUC gene family shows evidence for heteropolymers. A major characteristic of mucin peptide domain assembly is the distribution of cysteine residues forming disulfide bridges (Figure 6.2) located primarily in the cysteine-rich, cystine-knot sequences and von Willebrand C and D domains located at N- and C-terminal ends of the mucins [2,8] on either side of the central variable number of tandem repeat (VNTR) domains, which carry the mucin glycan chains. The sequence found in the major human, secreted intestinal mucin, MUC2, is as follows; from the N-terminus von Willebrand D1, D2, D’D3, cysteine-rich D, small PTS (Proline, Threonine, Serine), cysteine-rich D, large PTS, C terminal von Willebrand D4, von Willebrand B, von Willebrand C and, finally, cysteine knot domain (CK) at the C-terminus [9]. Figure 6.2 General mucin structure. (a) Topographical map of a human ocular mucin imaged with an atomic force microscope in HEPES buffer. This polymer, taken from a 500 nm2 area image scanned at 512 × 512 pixels, is longer than 3 μm, and of height between 0.66 and 1.5 nm. The small molecular diameters confirm regions of sparse glycosylation (0.66 nm is consistent with helical amino acids in a chain) and regions of dense, short glycans. (b) Schematic of a secreted mucin monomer; sequences similar to von Willebrand factor (D1–D3 at the N terminus) are involved in polymerisation, as is the cysteine knot domain (CK) at the carboxylic end of the mucin monomer. The cysteine domains (Cys) establish disulfide bonds between mucin polymers. The different number and location of these regions affect the pore size of gels formed by each mucin, and the heterogeneity of pore sizes in most mucus gels. After translation, dimerisation of MUC2 occurs in the endoplasmic reticulum [10], with subsequent mucin type O-glycosylation of the PTS domains in the Golgi apparatus. Trimer formation occurs in the trans-Golgi network [11] and these MUC2 forms are packed in the goblet cell granules. The molecular events that govern both granule storage and eventual secretion are analogous to those reported for von Willebrand factor oligomerisation and are dependent on vesicle pH and Ca2+ ion concentration [12]. The formation of MUC2 trimers is essential to facilitate the generation of mucus networks at the cell surface [12] and also provides a model to account for the dramatic increase in volume seen during mucin secretion (Figure 6.3). Figure 6.3 Polymerisation of MUC2. Asymmetry between C and N terminal polymerisation results in hexagonal arrangements. (a) Hexagonal array of MUC2; (b) linear dimers formed by C-C polymerisation; (c) trimers formed by the von Willebrand factor domains at the N-termini of MUC2 monomers. Redrawn from [12]. The membrane-associated mucins comprise major elements of the glycocalyx in the apical cell membranes on mucosal surfaces and have a peptide domain structure that reflects their functions at this location. They are monomeric, have a membrane spanning domain and do not form gels [8,13–16]. The characteristic, functional peptide domains found include the sea urchin sperm protein, enterokinase and agrin (SEA) module and epidermal growth factor (EGF)-like domain [17] (Figure 6.4). The SEA module has a peptide cleavage site which generates a noncovalent complex [18] and releases the large extracellular mucin fragment, which can ultimately be distinguished in the secreted mucus gel layer [19–21]. A further group of secreted soluble mucins is formed due to alternative splicing of MUC1 and these mucins have no transmembrane or cytoplasmic peptide domains [22,23]. These small mucin forms are not isolated with the typical, large mucins and thus require different methods for both isolation and detection. The membrane associated mucins in the glycocalyx present an O-glycan glycoarray at the cell surface and available for many host interactions occurring in the external milieu. Figure 6.4 Cell surface-associated mucins. (a) MUC4 deposited on graphite (McMaster et al., unpublished results). Note the accumulation of molecules on steps on the graphite where charge is not balanced. (courtesy of Kermit Carraway.) (b) Schematic of cell surface associated mucin: a. transmembrane domain (this may have a cytoplasmic GPI-anchor); b. mucin domain with O-linked oligosaccharides [*: N-linked oligosaccharides; grey: SEA domain detailed in insert (c)]. (c) The SEA domain in three cell surface mucins: in MUC4 this domain is replaced by epithelial growth factor receptor domains (grey); not all SEA domains in MUC16 are targeted by sheddases, proteolytic enzymes that cleave the mucin domain off the cell membrane. The characteristic glycosylation with mucin type O-glycans is located in the central, VNTR/PTS domains. The glycan chains are linked to serine and threonine residues via N-acetyl-D-Galactosamine, as part of a discrete number of core units. Eight different core structures have been identified but cores 1–4 are the most common [6,24–28]. The core units are enlarged by N-acetyl-lactosamine units and terminated with fucose, sialic acid or ester sulfate. N-linked glycans are also found but there much fewer chains and they are involved in the processing and subcellular localisation of the mucin glycopeptide during biosynthesis [29,30]. Additional post-translational mucin modifications include C-mannosylation of the CysD domains. This is an unusual carbon–carbon linkage of an α-manno-pyranosyl residue to the C2 indole carbon atom of the first Tryptophan in WXXW codons [31]. The role of C-mannosylation is believed to be in protein folding, subcellular localisation and trafficking levels at the Endoplasmatic Reticulum (ER)–Golgi interface [32,33]. The number of Cys domains varies between the secreted mucins with MUC2 having two, MUC5B 7 and MUC5AC nine copies and they are also found in other mammalian glycoproteins [2]. C-mannosylation of the Cys domains is necessary to enable normal maturation and secretion of mucins and acts as a signal for exit from the ER. Failure of this process leads to ER stress. The pathways of O-glycosylation have been well described and are expressed on a tissue-specific basis [5–7,24–28]. Glycosylation is also adapted at tissue locations. MUC2 has characteristic but variable glycosylation along the intestinal tract with discrete patterns identified from the small intestine through to the rectum. Oligosaccharides based on core 3 structures, GlcNAc(β1-3)GalNAc-R, comprise the majority of glycans found. Highly fucosylated glycans were located specifically in the small intestine, while sulfo-Lex carrying core 2 glycans occurred in the distal colon. Blood group H and A glycans were present exclusively in the ileum and caecum, and blood group Sda related epitopes showed an increasing gradient along the length of the colon. Furthermore, the mucin glycans contain an increasing gradient of sialic acid from the ileum to the colon associated with a decrease in fucose. The sialic acids in a heterogenous population of glycans showed considerable variations in the degree of O-acetylation [34,35]. The short glycans present on the ocular surface are a further example of adaptation to local physiology: in man, dog and rabbit these glycans are 3–5 sugars long [36–39]. Most glycans are negatively charged and terminate in sialic acids in humans, whereas in dog and rabbit they are mostly neutral, terminated in α1-2 fucose and/or α1-3 N-acetylgalactosamine (Figure 6.5). Figure 6.5 Proportions of glycans found in ocular mucin of dog, human and rabbit (data from [39]). Glycans not detectable by methods used here are graphed as 0.1% to emphasise their absence. SialylTn, not detected here in humans, is a common epitope by immunohistochemistry and immunoblotting in human ocular mucins. The diameter of individual mucin polymers varies in different organs: on the ocular surface, where glycans are at most five sugars long [40], the diameter of hydrated mucins is around 2 nm (measured with atomic force microscopy [38]). Gastric or bronchial mucins with much longer glycans have diameters of 7–10 nm (measured by negative shadowing [41]). It is well known that disease influences glycosylation. Infection and inflammation cause glycan modifications with changes that increase vulnerability to pathogens [5,7,42–44]. Examination of gastrointestinal disease has resulted in a number of biomarkers being identified, which map onto the relevant metabolic pathways [4,5,7,24,26–28]. MUC5AC is expressed in both stomach and trachea, with characteristic glycosylation in each tissue. Tracheal mucins showed about 50% neutral and 50% acidic with sialylated and sulfated structures. Neutral structures were largely based on core 1 and 2 and contained blood group H and Lewis type sequences, largely type 2 chains [Gal β1–4GlcNAc β1-] as Lewisx and Lewisy. Sialyated structures were both α2–3 and α 2–6 on core 1 and 2 with up to eight monosaccharides [45]. However, the glycoforms detected in gastric cancer carry truncated glycan chains [46]. The same pathological mechanisms lead to the altered MUC5AC glycosylation found in respiratory diseases such as cystic fibrosis (CF) and chronic bronchitis. These glycans normally neutral and highly sialylated and sulfated units with sizes ranging from 3–15 monosaccharides [47–49]. Alteration of mucin O-linked glycosylation leading to the development of gastric cancer has been linked with Helicobacter pylori infection [50]. The inflammation triggered by the bacteria modifies the host mucosal barrier glycan expression and, thus, the interaction with the bacteria. The induced glycans have been found to correlate with cancer cell infiltration and metastatic progression and are used as clinical biomarkers for gastric cancer progression. Screening for the O-glycans SLea (CA19-9) and STn (CA72), located on glycoproteins, including mucins, released from tumour cells, in patient blood samples is currently employed [50]. An N-glycosylation biomarker has also been reported in gastric cancer patient sera [51]. Identification of these glycan structures indicates the mechanism of disease progression in gastric carcinogenesis. A further example exists for breast cancer screening where a glycosylated MUC1 epitope found in cancer has been found to be more effective than the commonly used CA15.3 antibody assay [52]. The mucosal surface-associated mucus gel is an essential feature of the innate defensive barrier and must be in place at all times to afford effective protection. Turnover of the layer is due to a variety of factors present in the external environment and leads to disruption and degradation of the barrier components. Under normal conditions, in vivo, a positive balance exists between de novo synthesis of intact mucus and degradation and elimination of degraded mucus. Mucin biosynthesis, polymerisation and network formation on secretion generate the gel layer, while disruption of the gel and enzymatic degradation of the glycan chains and peptide backbone mediate turnover. The generation and cleavage of disulfide bonds linking the mucin monomers, dimers, trimers and oligomers are fundamental occurrences in the formation of mucin gel networks [8,12,53–57]. The creation and maintenance of molecular cross-links in mucus gels also occurs through the action of other mucosal proteins, including the trefoil peptides, gastrokines, transferrin and secretory IgA amongst others [28,58]. The different phases of mucin biosynthesis and secretion occur on variable time scales. MUC5AC biosynthesis takes place in approximately two hours [59], while secretion and hydration occur in the millisecond to second timescales [60]. Granular packing of mucins is established at pH 5.2 (in contrast to the higher values observed in the ER (pH 7.2) and trans-Golgi network (pH 6.0) in the presence of a high intragranular Ca2+ level [12]. The large increase in mucin volume accompanying secretion may be due to an ionic gradient where two monovalent Na+ ions are exchanged for divalent Ca2+ [12,56]. Adaptation of the mucosal barrier at specific organ and tissue sites is illustrated by mucin glycoforms of the same gene product in the same gland [61] or in adjacent goblet cells [62]. Glycoforms are populations of same gene product polymers with varying glycosylation, which results in different subunit charges. MUC5AC glycoforms are found in human ocular mucins purified from cadaver conjunctivae [63]; glycoforms or higher negative charge have been detected in MUC5AC deposited on contact lenses of asymptomatic wearers [64]. Different MUC gene products are synthesised at different tissue sites within the same organ, such as MUC5AC and MUC6 in the stomach, where discrete layers of each mucin can also be detected in the secreted mucus gel [65]. Cell surfaces are decorated with glycosylated molecules, some of which take part in adhesion, intercellular recognition and signal transduction. Apical surfaces of epithelial cells may be further covered with a glycocalyx that contains the extracellular, mucin, subunits of cell-surface associated mucins along other glycan-bearing molecules, such as mucopolysaccharides. It is widely held that the glycocalyx anchors the overlaying mucus gel to the surface of the epithelium and lubricates the cell surface. The extracellular domains of membrane-spanning mucins can be proteolytically cleaved or shed and thereafter integrate into the mucin gel formed mainly by the secreted mucins. Shedding provides, for example, for the renewal of the preocular fluid after sleep under the influence of neutrophil elastase [66] but occurs continuously as shown by the presence of surface-tethered mucins in open eye (waking) tears [67,68]. In perfused human colon biopsies the mucus layer was measured at 450 ± 70 μm, with a spontaneous growth of 240 ± 60 μm/h. A cholinergic agonist, Carbachol, adds 140 ± 80 μm over 30 minutes, most of which occurs in the first 15 minutes [69]. These substantial rates of growth and stimulated growth suggest that the thickness of the mucosa is regulated by various physiological mechanisms and is not yet fully understood. The elastic (G′) and viscous (G″) moduli and their relative magnitudes describe the behaviour of fluid under external forces and pinpoint sol–gel transitions. The elastic modulus G′ increases as more chains become involved in the gel. G″, the viscous modulus, can be taken to represent noninteracting molecular regions, adopting a random conformation. Flow and re-annealing can be explained by changes in the number and position of transient associations of these regions with the permanently linked domains of the gel [70]. A number of structural features of (secreted) mucins underpin mucous gels: secreted mucins are very long (of the order of microns) polyelectrolytes, therefore expected to form gels in the presence of cations in solution [71]. A mechanism that might be expected to dominate gelling between long polymers is entanglement. That this is not the only mechanism of gel formation is suggested by the absence of flow at low frequency stresses as expected for a purely entangled system [70]. Interactions between mucins occur through hydrogen bridges, through cations (e.g. Ca2+), as well as between hydrophobic regions of the protein cores, and between cysteines in these cores. Reducing disulfide bonds causes an almost instantaneous dissolution of the gel [72], as expected from polymer structure and the use of dithiothreitol to solubilise even the strongly aggregated mucins of cystic fibrosis patients [73]. Mucous gels contain other molecules, for example nucleic acids, peptides, or lipids that might contribute to gel formation and mucous gel properties. Trefoil factor peptides, present in all mucin gels studied, and which alter the persistence lengths of mucins (Brayshaw et al., unpublished), have been shown to alter the viscoelastic properties of mucin gels [74]; gel formation can however proceed in their absence. For gastric mucins, gelling is strongly dependent on acidic pH. At low mucin concentrations, mucin molecules in solution are not associated. At high concentration (10 mg/ml) and neutral pH, hydrodynamic interactions increase between segments of macromolecular chains in partially interpenetrating aggregates that are still in solution [75], while at pH 2 Text there is a vast increase in viscosity as hydrophobic domains act as cross-links between molecules in the gel. Helicobacter pylori, a clinically important gastric pathogen, gains motility through the gastric gel by increasing the pH, and thus decreasing the viscosity of the fluid in its vicinity, that is by effecting a local gel–sol transition [76]. It is not clear whether lipids are part of mucus gels or mere ‘contaminants’ due to the breakdown of cell membranes. Interactions between lipid micelles (or liposomes) and mucins are governed by the charge at the surface of the lipid layer [77]. However, whether single lipid molecules interact with gelled mucins is unclear, and so are the consequences of these interactions on the biophysical characteristics of the gel. Interactions between gall bladder lipids and mucins are well recognised: mucins bind lipids and form the matrix for cholesterol biliary stones [14,78]. Tears are an exception to lipid-poor mucosal gels, because a lipid layer covers the preocular mucus gel (and substantially lowers evaporation). The phospholipid transfer protein present in tears can be identified in immunoprecipitated tear mucins, and therefore understood to scavenge lipids interacting with these mucins [79]. Transfer of lipid ‘contaminated’ mucins away from the ocular surface might be the role of this protein, if lipid–mucin aggregates are not compatible with the tear film; equally, the phospholipid transfer protein might be bridging between the lipid and mucins as part of the tear film structure. From stomach to colon, the mucus gel covering the gastrointestinal tract changes nature and layering (Figure 6.6). MUC2 is the predominant mucin in the colon, and forms two distinct layers: one, towards the lumen, which is relatively loose and home to commensal bacteria, and the other, close to the epithelial surface that is dense and impenetrable to bacteria in healthy individuals. The ability of this mucin to form stacked gel layers follows from its structure and, in particular, because of the distribution of Cys domains in the molecule. This distribution allows MUC2 to form calcium-dependent trimers at its N-terminal, and dimers through its cysteine knot domains at the C-terminal. In the secreted molecules, layers of nets of hexameric rings are linked by the extended molecules that form parallel mucin bundles [12,32,55]. These layers, likely stabilised by interglycan interactions, have been observed in the dense part of the colonic gel. The looser gel that accommodates bacteria might arise from the action of bacterial enzymes, for example glycosidases that degrade the oligosaccharide chains sugar by sugar. It is tempting to think that the clear boundary between the dense and loose layers is a consequence of an equilibrium between rates of bacterial enzymatic activity and host gel secretion.
Structure and Properties of Mucins
6.1 Introduction
6.2 General Characteristics of Mucins
6.2.1 Mucin Genes and Gene Organisation
Mucin
Chromosome
Tandem repeat size (amino acids)
Secreted mucins – gel forming
MUC2
11p15.5
23
MUC5AC
11p15.5
8
MUC5B
11p15.5
29
MUC6
11p15.5
169
MUC19
12q12
19
Secreted mucins – nongel forming
MUC7
4q13 – q21
23
MUC8
12q24.3
13/41
MUC9
1p13
15
Membrane associated
MUC1
1q21
20
MUC3A/B
7q22
17
MUC4
3q29
16
MUC12
7q22
28
MUC13
3q21.2
27
MUC15
11p14.3
none
MUC16
19p13.2
156
MUC17
7q22
59
MUC20
3q29
18
MUC21
6p21
15
MUC22
6p21.3
10
6.2.2 Mucin Molecules, Structure and Organisation
6.3 Mucin Glycosylation – Changes in Disease
6.4 Dynamics of Mucin Synthesis and Function
6.5 Mucin Gel Formation on Cell Surfaces
6.5.1 Intermolecular Interactions in the Gel
6.5.2 Lipid Interactions
6.5.3 Layers in the Mucus Gel
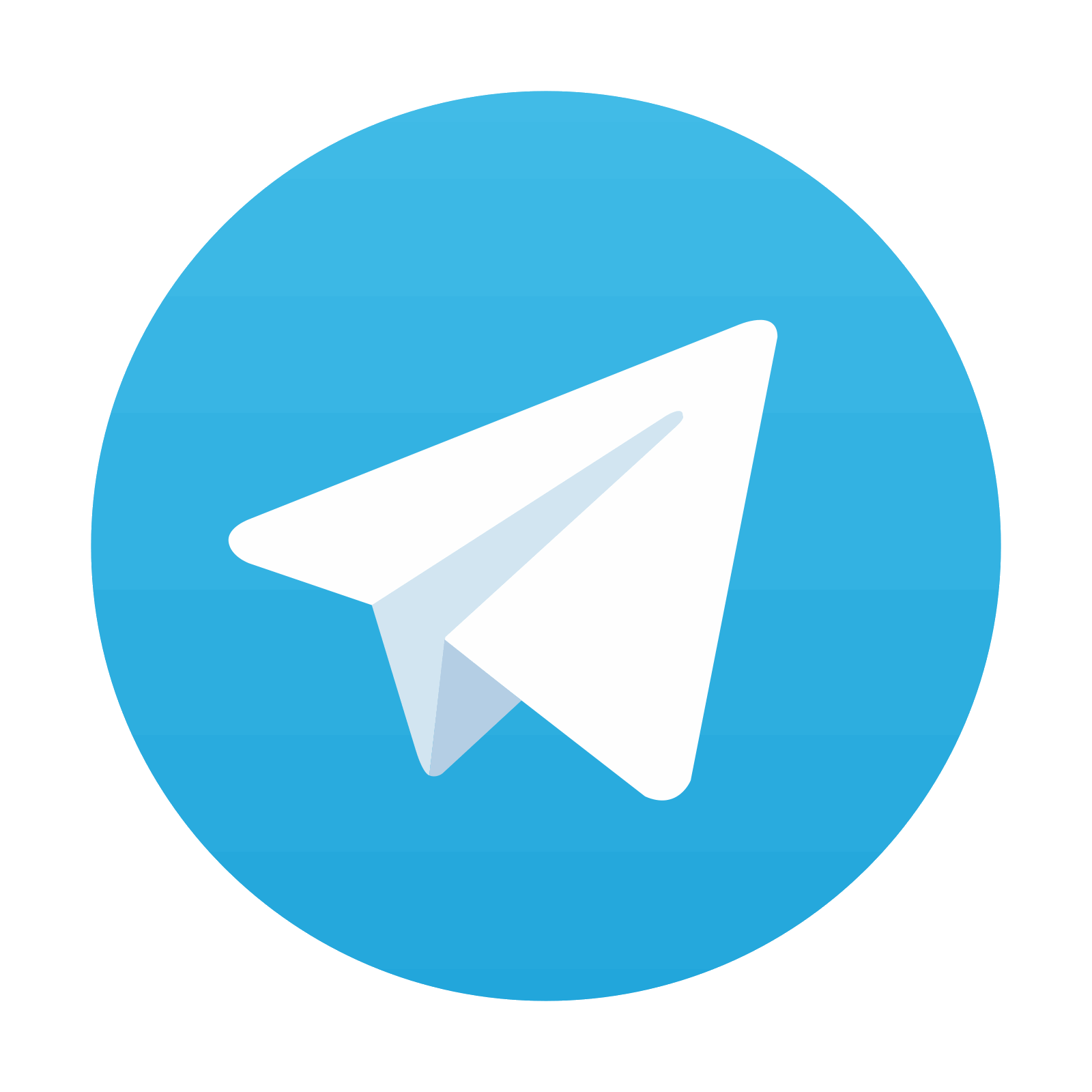
Stay updated, free articles. Join our Telegram channel
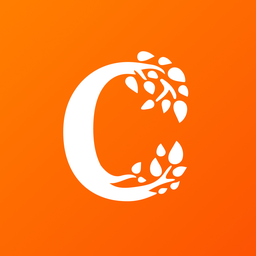
Full access? Get Clinical Tree
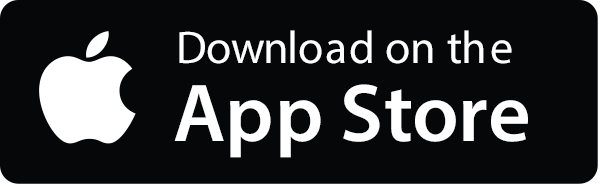
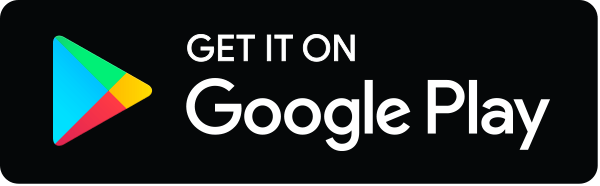