Chapter 37
Structure and Function of the Renal and Urologic Systems
Alexa K. Doig and Sue E. Huether
The primary function of the kidney is to maintain a stable internal environment for optimal cell and tissue metabolism. The kidneys accomplish these life-sustaining tasks by balancing solute and water transport, excreting metabolic waste products, conserving nutrients, and regulating acids and bases. The kidney also has an endocrine function, secreting the hormones renin, erythropoietin, and 1,25-dihydroxy-vitamin D3 for regulation of blood pressure, erythrocyte production, and calcium metabolism, respectively. The kidney also can synthesize glucose from amino acids, performing the process of gluconeogenesis (see What’s New? The Kidney and Glucose Regulation). The formation of urine is achieved through the processes of filtration, reabsorption, and secretion by the glomeruli and tubules within the kidney. The bladder stores the urine that it receives from the kidney by way of the ureters. Urine is then removed from the body through the urethra.
Structures of the Renal System
Structures of the Kidney
The kidneys are paired organs located in the posterior region of the abdominal cavity behind the peritoneum (Figure 37-1). They lie on either side of the vertebral column with their upper and lower poles extending from approximately the twelfth thoracic to the third lumbar vertebrae. The right kidney is slightly lower than the left and is displaced downward by the overlying liver. Each kidney is approximately 11 cm long, 5 to 6 cm wide, and 3 to 4 cm thick. A tightly adhering capsule (the renal capsule) surrounds each kidney, and the kidney then is embedded in a mass of fat. The capsule and fatty layer are covered with a double layer of renal fascia, fibrous tissue that attaches the kidney to the posterior abdominal wall.
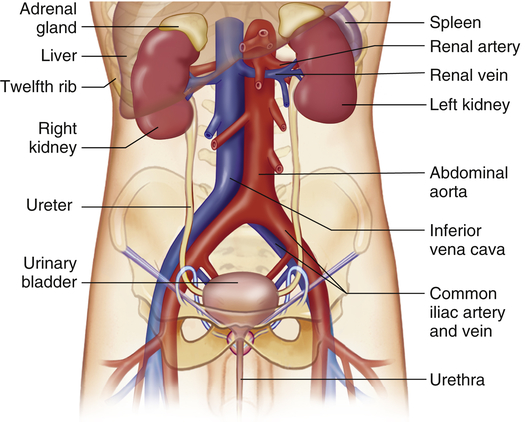
The structures of the kidney are summarized in Figure 37-2. The outer layer of the kidney is called the cortex and contains all of the glomeruli, most of the proximal tubules, and some segments of the distal tubule. The medulla forms the inner part of the kidney and consists of regions called the pyramids. Renal columns extend from the cortex down between the renal pyramids. The apexes of the pyramids project into minor calyces (cup-shaped cavities) that unite to form major calyces. The minor calyces receive urine from the collecting ducts through the renal papilla. The major calyces join to form the renal pelvis, which connects with the proximal end of the ureter. The walls of the calyces, pelvis, and ureter are lined with epithelial cells and contain smooth muscle cells that contract to move urine to the bladder.
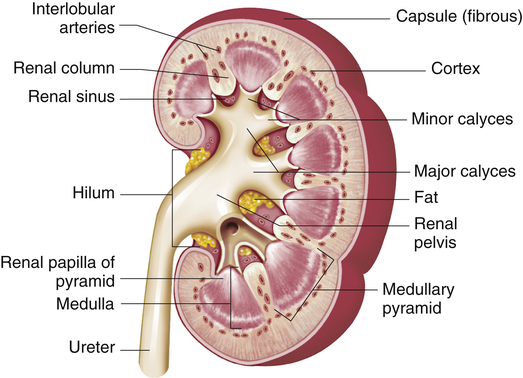
Nephron
The nephron is the functional unit of the kidney. Each kidney contains approximately 1.2 million nephrons. The nephron is a tubular structure with subunits that include the renal corpuscle, proximal convoluted tubule, loop of Henle (nephron ansa), distal convoluted tubule, and collecting duct, all of which contribute to the formation of urine (Figure 37-3). The different epithelial cells lining various segments of the tubule facilitate the special functions of reabsorption and secretion (Figure 37-4).
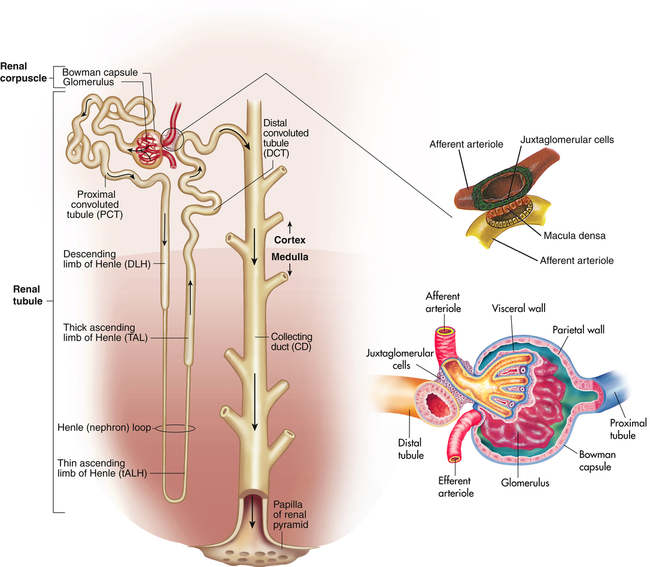
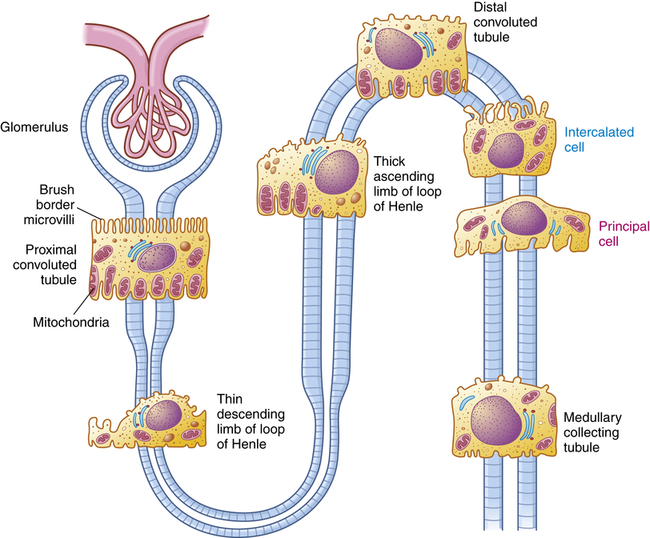
The brush border and high number of mitochondria in the cells of the proximal convoluted tubule permit reabsorption of 60% of the glomerular filtrate. Intercalated cells (blue text) secrete either H+ (reabsorb


The kidney has three kinds of nephrons: (1) superficial cortical nephrons (85% of all nephrons), which extend partially into the medulla; (2) midcortical nephrons with short or long loops; and (3) juxtamedullary nephrons, which lie close to and extend deep into the medulla and are important for the process of concentrating urine (Figure 37-5). The glomerulus (Figure 37-6; see also Figure 37-3) is a tuft of capillaries that loop into the circular Bowman glomerular capsule (Bowman space), like fingers pushed into bread dough. Mesangial cells and the mesangial matrix, secreted by mesangial cells, lie between and support the glomerular capillaries. Different mesangial cells contract like smooth muscle cells to regulate glomerular capillary blood flow. They also have phagocytic properties similar to monocytes and release inflammatory cytokines and growth factors.1 Together, the glomerulus, Bowman capsule, and mesangial cells are called the renal corpuscle.
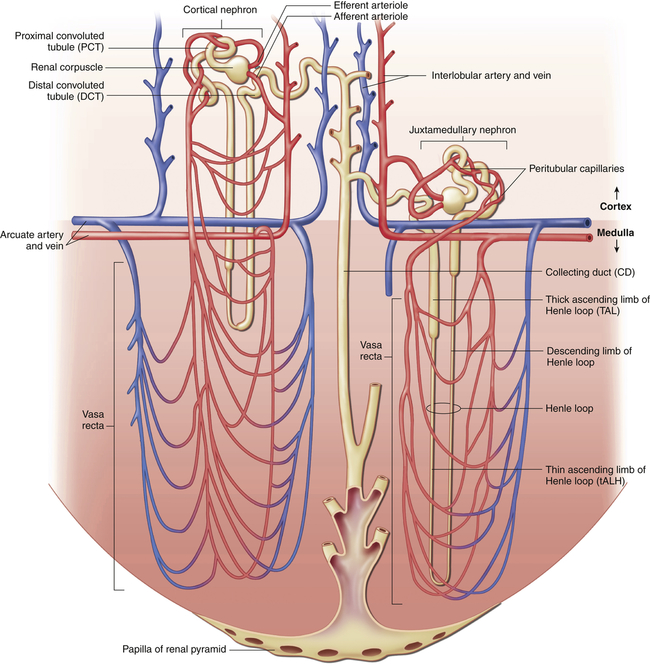
Blood flows through nephron vessels as follows: interlobular artery, afferent arteriole, glomerulus, efferent arteriole, peritubular capillaries (around the tubules), venules, interlobular vein. (From Patton KT, Thibodeau GA: Anatomy & physiology, ed 8, St Louis, 2013, Mosby.)
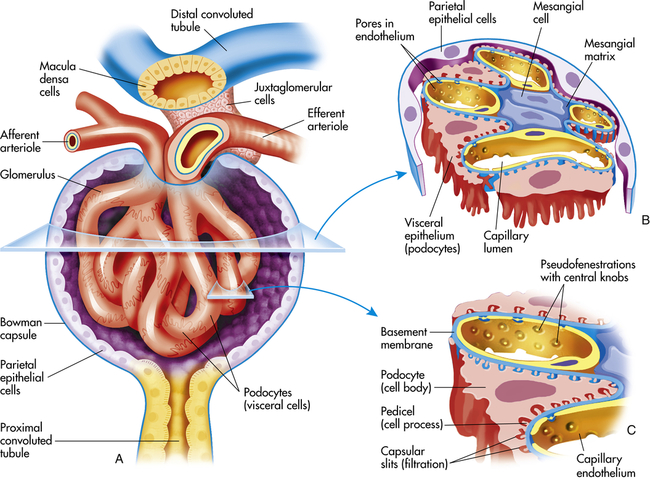
A, Longitudinal cross section of glomerulus and juxtaglomerular apparatus. B, Horizontal cross section of glomerulus. C, Enlargement of glomerular capillary filtration membrane.
The glomerular filtration membrane filters selected blood components through its three layers: (1) the inner layer is the glomerular endothelium, (2) the middle layer is the glomerular basement membrane (GBM), and (3) the outer layer is the visceral epithelium, which forms the inner layer of Bowman capsule. Each layer has unique structural properties that allow all components of the blood to be filtered, with the exception of blood cells and plasma proteins with a molecular weight greater than 70,000 (Figure 37-7; see also Figure 37-6).
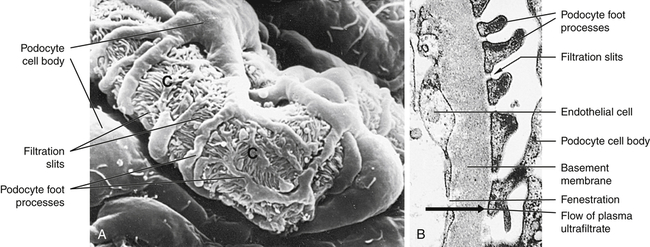
A, Scanning electron micrograph of normal glomerular capillary (C). B, Glomerular capillary wall (filtration membrane) (× 40,000). Black arrow indicates direction of urine flow. (From Kissane JM, editor: Anderson’s pathology, ed 9, St Louis, 1990, Mosby.)
Glomerular endothelial cells synthesize nitric oxide (a vasodilator) and endothelin-1 (a vasoconstrictor) that help regulate glomerular blood flow. The glomerular endothelium is perforated by many small openings or windows, called fenestrae. The fenestrae are maintained by vascular epithelial growth factor (VEGF) produced by the visceral epithelium. The middle basement membrane is composed of a selectively permeable network of proteoglycans (type IV collagen) secreted and maintained by the epithelial cells.2 The visceral epithelium of the Bowman capsule is composed of cells called podocytes (foot-like processes)3 that radiate and adhere to the basement membrane covering the glomerular capillaries. The foot processes of one podocyte interlock with the foot processes of adjacent podocytes, forming an elaborate network of intercellular clefts. These clefts are called filtration slits (see Figure 37-7) and modulate filtration. Nephrin, podocin, CD2-associated protein, and other protein molecules ensure proper function of the fenestrae.4
The glomerulus is supplied by the afferent arteriole and drained by the efferent arteriole. A group of specialized cells known as juxtaglomerular cells are located around the afferent arteriole where it enters the glomerulus (see Figures 37-3 and 37-6). Between the afferent and efferent arterioles is a portion of the distal convoluted tubule with specialized sodium- and chloride-sensing cells known as the macula densa. Together the juxtaglomerular cells and macula densa cells form the juxtaglomerular apparatus (JGA) (see Figure 37-6). Control of renal blood flow, glomerular filtration, and renin secretion occurs at this site.
The proximal convoluted tubule continues from the Bowman capsule and has an initial convoluted segment (pars convoluta) and then a straight segment (pars recta) that descends toward the medulla (see Figure 37-3). The wall of the tubule consists of one layer of cuboidal epithelial cells with a surface layer of microvilli (a brush border) that increases reabsorptive surface area. This is the only surface inside the nephron where the cells are covered with microvilli (see Figure 37-4). The proximal convoluted tubule joins the hairpin-shaped loop of Henle. The loop is composed of a thin descending segment, thin ascending segment and a thick ascending segment. The thin descending segment is composed of squamous cells, and is highly permeable to water but less permeable to ions. It has no active transport functions. The thin ascending segment is permeable to ions but not to water. The thick ascending segment actively transports ions into the interstitium and passes urine into the distal convoluted tubule (see Figure 37-14).
The distal convoluted tubule extends from the distal portion of the loop of Henley to the collecting duct. The collecting duct is a large tubule that descends down the cortex through the renal pyramids of the inner and outer medullae, draining urine into the minor calyx. The collecting duct is composed of two epithelial cell types: principal cells that reabsorb sodium and water and secrete potassium, and intercalated cells that secrete hydrogen and reabsorb potassium (see Figure 34-4).
Blood Vessels
The blood vessels of the kidney closely parallel nephron structure. The renal arteries arise from the abdominal aorta at the first lumbar vertebra and supply approximately 20% of the cardiac output to the kidneys. At the renal hilum they divide into anterior and posterior branches and then subdivide into lobar arteries that supply blood to the lower, middle, and upper portions of the kidney. The interlobar arteries are further subdivisions that travel down the renal columns and between the pyramids. At the cortical-medullary junction, interlobar arteries branch into the arcuate arteries that arch over the base of the pyramids and run parallel to the surface of the kidney. The interlobular arteries arise from the arcuate arteries and extend through the cortex toward the periphery of the kidney and supply the afferent glomerular arterioles (see Figure 37-5).
The afferent arterioles subdivide into the glomerulus, a fistlike structure of four to eight glomerular capillaries (see Figure 37-6). The glomerular capillaries feed into the efferent arteriole, which conveys blood to a second capillary bed, the peritubular capillaries. This is the only place in the body where an arteriole is positioned between two capillary beds. Increases or decreases in the resistance of the afferent and efferent arterioles increase or decrease glomerular filtration. The peritubular capillaries surround the the proximal and distal convoluted tubules and the loop of Henle (see Figure 37-5). The peritubular capillaries are adapted differently for the cortical and juxtamedullary nephrons. The peritubular capillaries surrounding the tubules of the cortical nephrons are similar to capillaries in other tissues. For the juxtamedullary nephrons a network of capillaries called the vasa recta forms loops and closely follows the loops of Henle. The capillaries of the vasa recta are the only blood supply to the medulla. They influence the osmolar concentration of the medullary extracellular fluid, which is important to the formation of concentrated urine. All capillaries then drain into the venous system. The renal veins follow the arterial path in a reverse direction and have the same names as the arteries. The renal vein empties into the inferior vena cava. The lymphatic vessels tend also to follow the distribution of the blood vessels.
Urinary Structures
Ureters
The urine formed by the nephrons flows from the distal tubules and collecting ducts through the duct of Bellini and the renal papillae (projections of the ducts) into the calyces, where it is collected in the renal pelvis (see Figure 37-2) and then funneled into the ureters. Each adult ureter is approximately 30 cm long and is composed of long, intertwining smooth muscle bundles. The lower ends of the ureters pass obliquely through the posterior aspect of the bladder wall. The close approximation of smooth muscle cells permits the direct transmission of electrical stimulation from one cell to another. The resulting downward peristaltic activity propels urine into the bladder. Contraction of the bladder during micturition (urination) compresses the lower end of the ureter, preventing reflux. Peristalsis is maintained even when the ureter is denervated, so ureters can be transplanted.
Bladder and Urethra
The bladder is a bag composed of a basket weave of smooth muscle fibers that forms the detrusor muscle and its smooth lining of transitional epithelium (uroepithelium). As the bladder fills with urine, it distends and the layers of transitional epithelial cells within the lining slide past each other and become thinner as the volume of the bladder increases. The transitional epithelium forms the interface between the urinary space and underlying vasculature, connective, nervous, and muscle tissue. It senses and transduces information about luminal pressure and urine composition within the urinary tract.5 The trigone is a smooth triangular area lying between the openings of the two ureters and the urethra (Figure 37-8). The position of the bladder varies with age and gender. In infants and young children the bladder rises above the symphysis pubis, providing easy access for percutaneous aspiration. In adults it lies in the true pelvis, in front of the rectum and in front of the uterus in women. Inferiorly, the bladder sits on the prostate in men and on the anterior vagina in women. The bladder has a profuse blood supply, accounting for the bleeding that readily occurs with trauma, surgery, or inflammation.
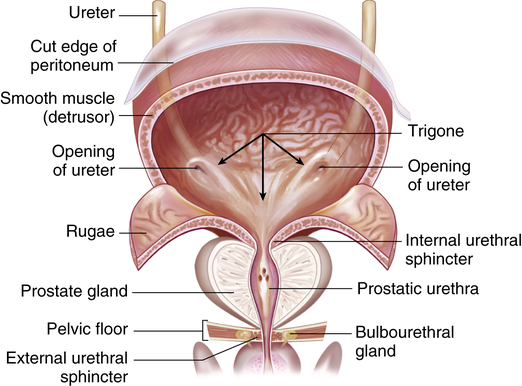
Frontal view of a dissected urinary bladder (male) in a fully distended position. (From Patton KT, Thibodeau GA: Anatomy & physiology, ed 8, St Louis, 2013, Mosby.)
Renal Blood Flow
Autoregulation of Renal Blood Flow
In the kidney a local mechanism of autoregulation tends to keep the rate of glomerular perfusion and therefore the GFR fairly constant over a range of arterial pressures between 80 and 180 mmHg (Figure 37-9). This means that changes in afferent arteriolar pressure and resistance occur in the same direction. For example, as systemic blood pressure increases, the afferent arterioles constrict, preventing an increase in filtration pressure. Opposite processes occur with a decrease in systemic blood pressure. Therefore, renal blood flow and GFR are relatively constant. The purpose of renal autoregulation is to prevent wide fluctuations in systemic arterial pressure from being transmitted to the glomerular capillaries. In this way, large fluctuations in GFR are prevented and solute and water excretion is constantly maintained despite arterial pressure changes.6 Autoregulation may also protect the kidney from damage by hypertension.
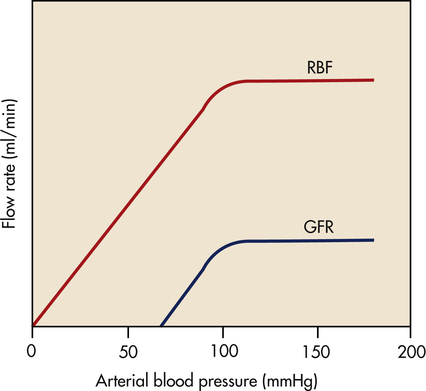
Blood flow and glomerular filtration rate are stabilized with changes in mean arterial pressure between about 80 and 180 mmHg. (From Berne RM, Levy MN, editors: Principles of physiology, ed 4, St Louis, 2006, Mosby.) GFR, Glomerular filtration rate; RBF, renal blood flow.
One mechanism responsible for the autoregulatory response in the kidney is a myogenic mechanism. As arterial pressure declines, the stretch on the afferent arteriolar smooth muscle decreases and the arteriole relaxes, causing an increase in glomerular perfusion; an increase in arteriolar pressure causes the arteriole smooth muscle to contract and decreases glomerular perfusion. Tubuloglomerular feedback is a second mechanism for keeping renal blood flow and GFR constant and stable. As the GFR in an individual nephron increases or decreases, the macula densa cells in the distal tubule sense the increasing or decreasing amounts of filtered sodium. When sodium filtration increases, the macula densa cells stimulate afferent arteriolar vasoconstriction to decrease GFR. The opposite occurs with decreases in sodium filtration at the macula densa.7 A third unknown mechanism also contributes to autoregulation.8
Neural Regulation
The blood vessels of the kidney are innervated by sympathetic adrenergic/noradrenergic fibers that cause arteriolar vasoconstriction to reduce renal blood flow. The innervation of the kidney comes primarily from the celiac ganglion and greater splanchnic nerve (see Figure 15-25). The afferent and efferent arterioles are richly innervated, but nerves have not been observed in the glomerular capillaries.
Hormones and Other Factors
Hormonal factors and many mediators can alter the resistance of the renal vasculature by stimulating vasodilation or vasoconstriction. A major hormonal regulator of renal blood flow is the renin-angiotensin-aldosterone system (RAAS), which can increase systemic arterial pressure and increase sodium reabsorption. Renin is an enzyme formed and stored in granular cells of the afferent arterioles of the JGA (see Figure 37-3). The release of renin is principally triggered by decreased blood pressure in the afferent arterioles, which reduces stretch of the juxtaglomerular cells; decreased sodium chloride concentrations in the distal convoluted tubule; sympathetic nerve stimulation of β-adrenergic receptors on the juxtaglomerular cells; and release of prostaglandins.9
When renin is released, it cleaves an α-globulin (angiotensinogen produced by liver hepatocytes) in the plasma to form angiotensin I, which is physiologically inactive. In the presence of angiotensin-converting enzyme (ACE) produced from the pulmonary and renal endothelium, angiotensin I is converted to angiotensin II. Angiotensin II stimulates secretion of aldosterone by the adrenal cortex (see Chapter 21), is a potent vasoconstrictor, and stimulates antidiuretic hormone (ADH) secretion and thirst. Vitamin D3 is a potent negative endocrine regulator of renin gene expression.10 Numerous physiologic effects of the RAAS serve the purpose of stabilizing systemic blood pressure and preserving the extracellular fluid volume during hypotension or hypovolemia, including sodium reabsorption, potassium excretion, systemic vasoconstriction, sympathetic nerve stimulation, thirst stimulation, and drinking. (The combined effects of the RAAS are summarized in Figure 37-10.) ACE inhibitors are a class of drugs that reduce blood pressure by inhibiting the formation of angiotensin II and aldosterone.
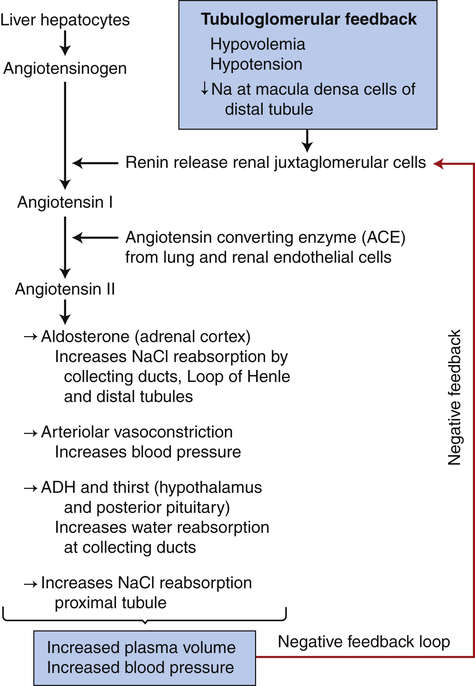
Activation of tubuloglomerular feedback mechanisms stimulates the release of renin with activation of the renin-angiotensin-aldosterone system. Plasma volume and blood pressure are increased with the reabsorption of sodium chloride and water from the renal tubules. The restoration of plasma volume and blood pressure then decrease the release of renin, forming a negative feedback loop. ADH, Antidiuretic hormone.
Natriuretic peptides are a group of peptide hormones, including atrial natriuretic peptide (ANP), secreted from myocardial cells in the atria and brain natriuretic peptide (BNP) secreted from myocardial cells in the cardiac ventricles. When the heart dilates during volume expansion or heart failure, ANP and BNP inhibit sodium and water absorption by kidney tubules, inhibit secretion of renin and aldosterone, vasodilate the afferent arterioles, and constrict the efferent arterioles. The result is increased urine formation leading to decreased blood volume and blood pressure.11 C-type natriuretic peptide is secreted from vascular endothelium and in the nephron and causes vasodilation.12 Urodilatin is secreted by the distal convoluted tubules and collecting ducts and causes vasodilation and natriuretic and diuretic effects. Other hormones and mediators that influence renal blood flow are summarized in Table 37-1.
TABLE 37-1
HORMONES, MEDIATORS, AND RENAL BLOOD FLOW
HORMONE OR MEDIATOR | EFFECT ON RENAL BLOOD FLOW |
Adenosine | Produced within kidney; causes vasoconstriction of afferent arteriole; decreases RBF and GFR |
Angiotensin II | Produced systemically and within kidneys; constricts afferent and efferent arterioles; decreases RBF and GFR |
Atrial and brain natriuretic peptides | Produced by atria and ventricles of the heart with hypertension and increased blood volume; causes vasodilation of afferent arteriole and vasoconstriction of efferent arteriole; modest increase in GFR with little change in RBF |
Bradykinin | Produced in kidney from kininogen and causes vasodilation by release of nitric oxide and prostaglandins; increases RBF and GFR |
Dopamine | Produced by the proximal tubule; increases RBF; inhibits renin secretion |
Endothelin | Produced by renal vessel endothelial cells, mesangial cells, and distal tubule cells in response to bradykinin, angiotensin II, epinephrine, and stretch; most active with renal disease; profound vasoconstriction of afferent and efferent arterioles; decreases RBF and GFR |
Histamine | Produced locally within the kidney; modulates RBF in basal state and during inflammation; increases RBF by decreasing afferent and efferent arteriolar resistance and does not decrease GFR |
Nitric oxide | Produced by renal vessel endothelial cells with increased stretch and by stimulation of acetylcholine, histamine, bradykinin, ATP; increases vasodilation of afferent and efferent arterioles |
Prostaglandins, PGI2, PGE2 | Produced locally within kidney with decreased RBF; dampen vasoconstriction caused by sympathetic nerves and angiotensin II; prevent harmful vasoconstriction and renal ischemia |
Urodilatin (a natriuretic peptide) | Produced by distal tubule and collecting duct when there is increased circulating volume and increased blood pressure; inhibits sodium and water reabsorption from medullary part of collecting duct, thereby producing diuresis |
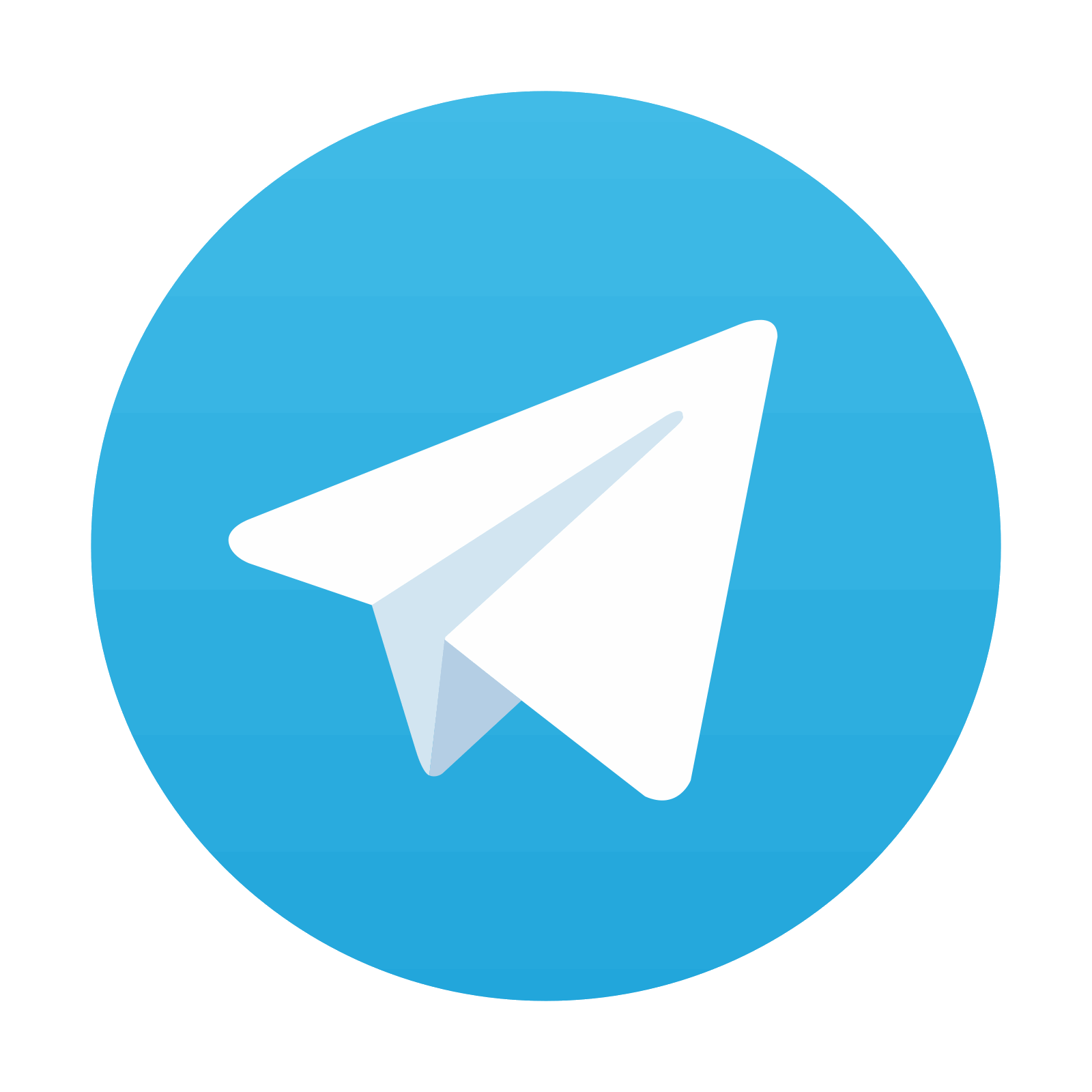
Stay updated, free articles. Join our Telegram channel
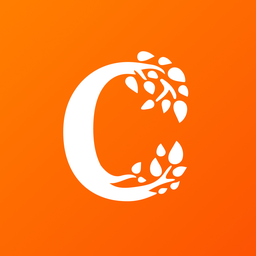
Full access? Get Clinical Tree
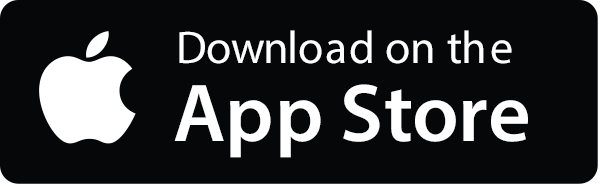
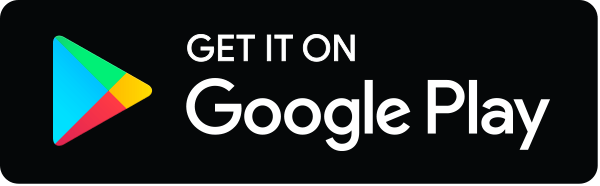