Chapter 15
Structure and Function of the Neurologic System
Cells of the Nervous System
The two basic types of cells that comprise nervous tissue are neurons and neuroglial cells. The neuron is the primary information/communication cell of the nervous system. Working in parallel systems, neurons can scan the environment, integrate many systems at higher cognitive levels, and initiate body responses to maintain homeostasis. The neuroglial cells are found in the CNS and PNS and can provide structural support and nutrition for neurons, remove debris, increase the speed of nerve impulses, and play a significant role, along with neurons, in processing and storing information (i.e., memory).1
Neurons
A neuron (Figure 15-1) has three components: a cell body (soma) and the thin processes of the cell—the dendrites and axons. Most cell bodies are located within the CNS. Dense, packed cell bodies in the CNS are called nuclei. Cell bodies in the PNS are usually found in groups called ganglia or plexuses. The dendrites are extensions that carry nerve impulses toward the cell body. The dendritic zone is the receptive portion of a neuron that receives a stimulus and continues further conduction. Axons are long, conductive projections from the cell body that carry nerve impulses away from the cell body. The axon hillock is the cone-shaped, organelle-free area where the axon leaves the cell body. In large nerves, axons are bundled together as fascicles. The initial segment of the axon has the lowest threshold for stimulation, and as a result, action potentials begin there.
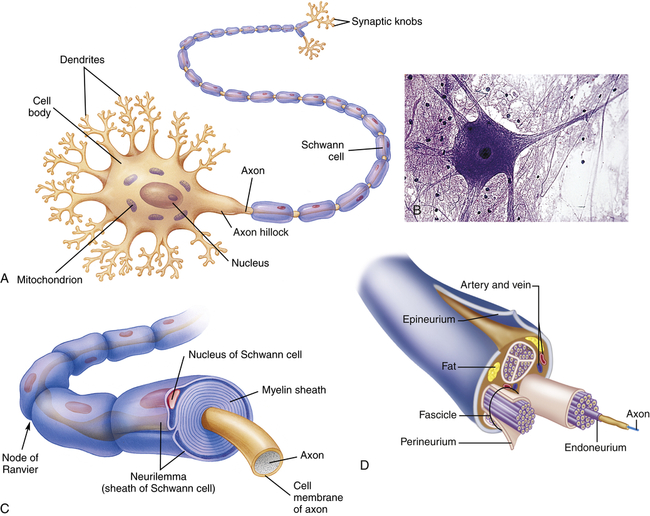
A, Many dendrites carry nerve impulses to the cell body, and from the cell body the nerve impulses are conducted along a single, long axon. Long axons are encased at intervals by a myelin sheath. B, Photomicrograph of a neuron. C, A segment of myelinated fiber in cross section, showing myelin sheath composed of several layers of myelin, which insulate the axon. D, Axons bundled into fascicles. (A and C from Thibodeau GA, Patton KT: Structure and function of the human body, ed 12, St Louis, 2004, Mosby; B, copyright Edward Reschke; D from Patton KT, Thibodeau GA: Anatomy & physiology, ed 8, St Louis, 2013, Mosby.)
A typical neuron has only one axon, which may be covered with a segmented layer of lipid material called myelin, which acts as an insulating substance. This entire membrane is referred to as the myelin sheath; the thin membrane between the myelin sheath and the endoneurium, a delicate connective tissue around each axon in the PNS (see Figure 15-1, C), is the neurilemma (Schwann sheath). The neurilemma and the myelin sheath are interrupted at regular intervals by the nodes of Ranvier. Myelin acts as an insulator that allows ions to flow between segments rather than along the entire length of the membrane, resulting in increased velocity of neuronal conduction. This mechanism is referred to as saltatory conduction. If the Schwann cells are loosely wrapped around the axon, it is referred to as unmyelinated, and conduction velocity is not increased. Axons are capable of extensive branching, which occurs at the nodes of Ranvier. Two major principles of information processing in the nervous system are divergence and convergence. Divergence refers to the ability of these branching axons to influence many different neurons. Convergence is the term applied to branches of numerous neurons converging on and influencing one or a few neurons. Disorders of the myelin sheath (demyelinating diseases), such as multiple sclerosis and Guillain-Barré syndrome, demonstrate the important role myelin plays in nerve function (see Chapter 18). Besides depending on the myelin coating, conduction velocities also are influenced by the diameter of the axon. Larger axons transmit impulses at a faster rate.
Neurons are structurally classified on the basis of the number of processes (projections) extending from the cell body. There are four basic types of cell configuration: (1) unipolar, (2) pseudounipolar, (3) bipolar, and (4) multipolar (Figure 15-2). Unipolar neurons have one process that branches shortly after leaving the cell body. One example is found in the retina. Pseudounipolar neurons (some authors call them unipolar) have one process that has its dendritic portion extending away from the CNS and its axon portion projecting into the CNS (see Figure 15-2, C). The configuration is typical of sensory neurons in both cranial and spinal nerves. Bipolar neurons have two distinct processes arising from the cell body. This type of neuron connects to rod and cone cells of the retina. Multipolar neurons are the most common and have multiple dendrites and a single axon. A motor neuron is typically multipolar.
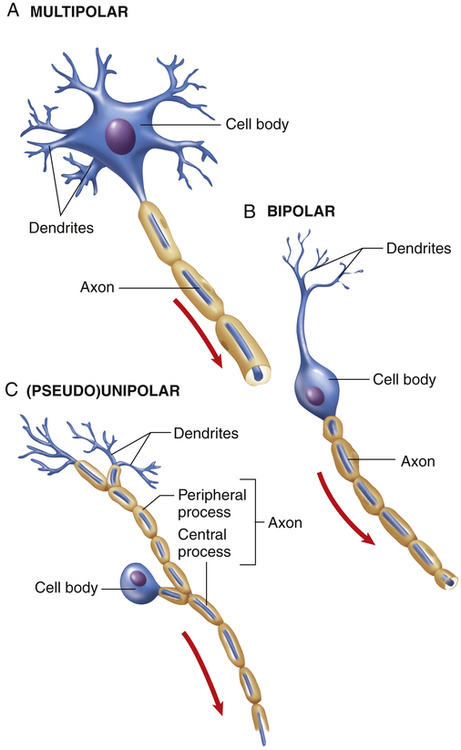
Functionally, there are three types of neurons (with their direction of transmission and typical configuration noted in parentheses): (1) sensory (afferent, mostly pseudounipolar), (2) associational (interneurons, multipolar), and (3) motor (efferent, multipolar). Sensory neurons carry impulses from peripheral sensory receptors to the CNS (Box 15-1). Association neurons (interneurons) transmit impulses from neuron to neuron, for example, from sensory to motor neurons, and are also involved in cognitive function. Motor neurons transmit impulses away from the CNS to an effector organ. In skeletal muscle the end processes form a complex neuromuscular (myoneural) junction.
Neuroglia and Schwann Cells
Neuroglia comprise the general classification of cells that support the neurons of the CNS. They make up approximately half of the total brain and spinal cord volume and are 5 to 10 times more numerous than neurons. Different types of neuroglia serve different functions. Astrocytes, for example, fill the spaces between neurons and surround blood vessels in the CNS (see What’s New? Astrocytes). Oligodendrocytes function to deposit myelin within the CNS. Oligodendroglia are the CNS counterpart of the Schwann cells. Ependymal cells line the cerebrospinal fluid (CSF)–filled cavities of the CNS. Microglia remove debris (phagocytosis) in the CNS. Characteristics and
functions of neuroglia and Schwann cells are summarized in Figure 15-3 and Table 15-1.
TABLE 15-1
SUPPORT CELLS OF THE NERVOUS SYSTEM
CELL TYPE | PRIMARY FUNCTIONS |
Astrocytes | Form specialized contacts between neuronal surfaces and blood vessels |
Provide rapid transport for nutrients and metabolites | |
Believed to form an essential component of the blood-brain barrier | |
Appear to be the scar-forming cells of the CNS, which may be the foci for seizures | |
Appear to work with neurons in processing information and memory storage | |
Oligodendroglia (oligodendrocytes) | Formation of myelin sheath and neurilemma in the CNS |
Schwann cells | Formation of myelin sheath and neurilemma in the PNS |
Microglia | Responsible for clearing cellular debris (phagocytic properties) |
Ependymal cells | Serve as a lining for ventricles and choroid plexuses involved in production of cerebrospinal fluid |
CNS, Central nervous system; PNS, peripheral nervous system.
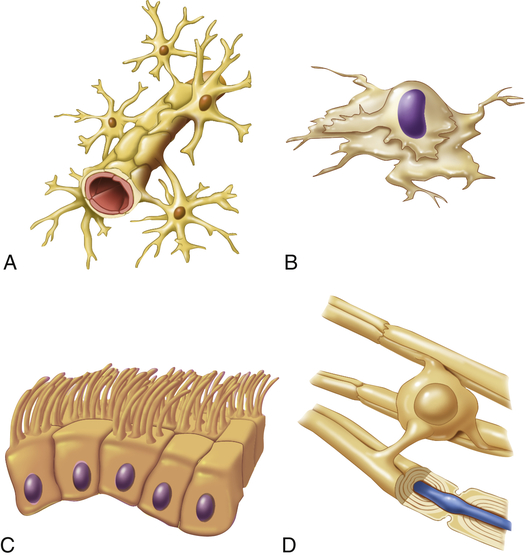
A, Astrocyte attached to brain capillary; B, microglial cell; C, ependymal cells that form sheets to line fluid cavities in brain; D, oligodendrocyte wrapped around CNS nerve fiber forming myelin. (From Patton KT, Thibodeau GA: Anatomy & physiology, ed 8, St Louis, 2013, Mosby.)
The Schwann cell, or neurolemmocyte, is a glial cell that wraps around and covers axons in the peripheral nervous system. Schwann cells form and maintain the myelin sheath, and the nodes of Ranvier form the spaces on either side of the Schwann cell. If the myelin layer is tightly wrapped many times around the axon forming nodes of Ranvier, it increases conduction velocity and the neuron is referred to as myelinated (see Figure 15-1).
Nerve Injury and Regeneration
At the proximal end of the injured axon, similar changes occur, but only back as far as the next node of Ranvier. The cell body responds to trauma by swelling and then dispersing the Nissl substance (chromatolysis). During the repair process the cell increases metabolic activity, protein synthesis, and mitochondrial activity. Approximately 7 to 14 days after the injury, new terminal sprouts project from the proximal segment and may enter the remaining Schwann cell pathway. (Figure 15-4 contains a representation of these events.) This process, however, is limited to myelinated fibers and generally occurs only in the PNS. The regeneration of axonal constituents in the CNS is limited by increased scar formation and the different nature of myelin formation by the oligodendrocyte.
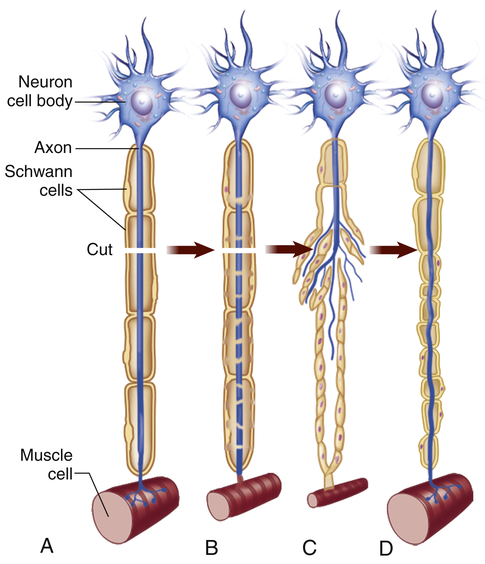
When cut, a damaged motor axon can regrow to its distal connection only if the neurilemma remains intact (to form a guiding tunnel) and if scar tissue does not block its pathway. (From Patton KT, Thibodeau GA: Anatomy & physiology, ed 8, St Louis, 2013, Mosby.)
Nerve Impulse
Neurons generate and conduct electrical and chemical impulses by selectively changing the electrical portion of their plasma membranes and influencing other nearby neurons by the release of chemicals (neurotransmitters). A neuron in its unexcited state maintains a resting membrane potential (see Chapter 1). When the membrane potential is raised sufficiently, an action potential is generated (see Figure 1-35), and the nerve impulse then flows to all parts of the neuron. The action potential response occurs only when the stimulus has sufficient strength; if it is too weak, the membrane remains unexcited. This property is sometimes termed the all-or-none response.
Synapses
Neurons are not physically continuous with one another. The region between adjacent neurons is called a synapse. Impulses are transmitted across the synapse by chemical (see Figures 15-5 and 15-14) and electrical conduction (Chapter 1); only chemical conduction is discussed here. The neurons that conduct a nerve impulse are named according to whether they relay impulses toward the synapse (presynaptic neurons) or away from the synapse (postsynaptic neurons). Four basic types of connections occur in regions of contact between the presynaptic and postsynaptic neurons. These are between axons (axo-axonic), from axon to cell body (axo-somatic), from axon to dendrite (axo-dendritic), and from dendrite to dendrite (dendro-dendritic).
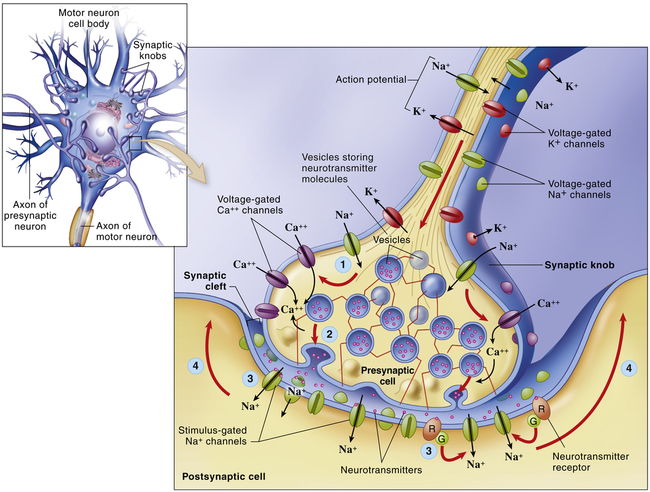
The electrical impulse travels along the axon of the first neuron to synapse at synaptic knobs. The chemical transmitter is secreted into the synaptic space to depolarize the membrane (dendrite or cell body) of the next neuron in the pathway. Details illustrate the synaptic knob (axon terminal) of a presynaptic neuron, the plasma membrane of a postsynaptic neuron, and a synaptic cleft. At step (1)—the arrival of an action potential at the synaptic knob—voltage-gated Ca++ channels open and allow extracellular Ca++ to diffuse into the presynaptic cell. At step (2) the Ca++ triggers the rapid exocytosis of neurotransmitter molecules from vesicles in the knob. At step (3) neurotransmitter diffuses into the synaptic cleft and binds to receptor molecules in the plasma membrane of the postsynaptic neuron. The postsynaptic receptors directly or indirectly trigger the opening of stimulus-gated ion channels, initiating a local potential in the postsynaptic neuron. At step (4) the local potential may move toward the axon, where an action potential may begin. (From Patton KT, Thibodeau GA: Anatomy & physiology, ed 8, St Louis, 2013, Mosby.)
Impulses are transmitted across the synapse by chemical conduction. The conducting substance is called a neurotransmitter and it is often formed in the neuron, transported to the synaptic knobs (boutons) of the presynaptic neuron’s axon, and stored in synaptic vesicles within the knobs. Action potentials in the presynaptic neuron cause the synaptic vesicles to release their neurotransmitter(s) through the plasma membrane into the synaptic cleft (the space between the neurons), where they bind to specific neurotransmitter (protein) receptor sites on the plasma membrane of the postsynaptic neuron (see Figure 15-5). Neurons can synthesize more than one neurotransmitter, and postsynaptic membranes can contain more than one type of transmitter-specific receptor.
Neurotransmitters
A neurotransmitter is defined as a chemical that “must be synthesized in the neuron, become localized in the presynaptic terminal (synaptic bouton), be released into the synaptic cleft, bind to a receptor site (binding site) on the postsynaptic membrane of another neuron or effector where it affects ion channels, and last, be removed by a specific mechanism from its site of action.” More than 46 neurotransmitters, including norepinephrine, acetylcholine, dopamine, histamine, gamma-aminobutyric acid (GABA), and serotonin, have been identified.2,3 Many of these transmitters have more than one function. For example, norepinephrine in the brain probably helps regulate mood, functions in dream sleep, and maintains arousal. Several neurotransmitters are amino acids, including GABA, glutamic acid, and aspartic acid. Small chains of amino acids, such as enkephalins and endorphins, also function as neurotransmitters. They (neuropeptides) are involved in the perception and integration of pain, as well as in emotional experiences. Neurotransmitter and neuromodulator substances are listed in Table 15-2.
TABLE 15-2
SUBSTANCES THAT ARE NEUROTRANSMITTERS OR NEUROMODULATORS
SUBSTANCE | LOCATION | EFFECT | CLINICAL EXAMPLE |
Acetylcholine | Many parts of the brain, spinal cord, neuromuscular junction of skeletal muscle, and many ANS synapses | Excitatory or inhibitory | Alzheimer disease (a type of dementia) is associated with a decrease in the number of acetylcholine-secreting neurons. Myasthenia gravis (weakness of skeletal muscles) results from a reduction in the number of acetylcholine receptors. |
Monoamines | |||
Norepinephrine | Many areas of the brain and spinal cord; also in some ANS synapses | Excitatory or inhibitory | Cocaine and amphetamines∗ result in overstimulation of postsynaptic neurons. |
Serotonin | Many areas of the brain and spinal cord | Generally inhibitory | Is involved with mood, anxiety, and sleep induction. Levels of serotonin are elevated in schizophrenia (delusions, hallucinations, withdrawal). |
Dopamine | Some areas of the brain and ANS synapses | Generally excitatory | Parkinson disease (depression of voluntary motor control) results from destruction of dopamine-secreting neurons. Drugs used to increase dopamine production induce vomiting and schizophrenia. |
Histamine | Posterior hypothalamus | Excitatory (H1 and H2 receptors) and inhibitory (H3 receptors) | There is no clear indication of histamine-associated pathologic conditions. Histamine is involved with arousal and attention and links to other brain transmitter systems. |
Amino Acids | |||
Gamma-aminobutyric acid (GABA) | Most neurons of the CNS have GABA receptors | Majority of postsynaptic inhibition in the brain | Drugs that increase GABA function have been used to treat epilepsy by inhibiting excessive discharge of neurons. |
Glycine | Spinal cord | Most postsynaptic inhibition in the spinal cord | Glycine receptors are inhibited by strychnine. |
Glutamate and aspartate | Widespread in brain and spinal cord | Excitatory | Drugs that block glutamate or aspartate, such as riluzole, are used to treat amyotrophic lateral sclerosis. These drugs might prevent overexcitation from seizures and neural degeneration. |
Neuropeptides | |||
Endorphins and enkephalins | Widely distributed in the CNS and PNS | Generally inhibitory | Morphine and heroin bind to endorphin and enkephalin receptors on presynaptic neurons and reduce pain by blocking the release of neurotransmitter. |
Substance P | Spinal cord, brain, and sensory neurons associated with pain, GI tract | Generally excitatory | Substance P is a neurotransmitter involved in pain transmission pathways. Blocking release of substance P by morphine reduces pain. |
∗Increase the release and block the reuptake of norepinephrine.
From Seeley R, Stephens TD, Tate P: Anatomy and physiology, ed 7, New York, 2006, McGraw-Hill.
Because the neurotransmitter is normally stored on one side of the synaptic cleft and the receptor sites are on the other side, chemical synapses operate in one direction. Therefore, action potentials are transmitted along a multineuronal pathway in one direction. The binding of the neurotransmitter at the receptor site changes the permeability of the postsynaptic neuron and, consequently, its membrane potential. Two possible scenarios can then follow: (1) the postsynaptic neuron may be excited (depolarized; excitatory postsynaptic potentials [EPSPs]), or (2) the postsynaptic neuron’s plasma membrane may be inhibited (hyperpolarized; inhibitory postsynaptic potentials [IPSPs]). Cannabinoid transmitters are released from postsynaptic neurons that modulate neurotransmitter release from presynaptic neurons.4,5 (Chapter 1 contains a review of electrical impulses and membrane potentials.)
Central Nervous System
Brain
The human brain enables individuals to reason, function intellectually, express personality and mood, and interact with the environment. The brain is a pinkish gray organ that weighs approximately 3 pounds and has the consistency of tofu or custard. It receives approximately 15% to 20% of the total cardiac output. The three major divisions of the brain, based on embryologic origin, are: (1) the forebrain, formed by the two cerebral hemispheres; (2) the midbrain, which includes the corpora quadrigemina, tegmentum, and cerebral peduncles; and (3) the hindbrain, which includes the cerebellum, pons, and medulla (Table 15-3). The midbrain, medulla oblongata, and pons make up the brainstem, which connects the hemispheres of the brain, cerebellum, and spinal cord. A collection of nuclei (nerve cell bodies) within the brainstem collectively constitute the reticular formation (Figure 15-6). The reticular formation is a large network of connected tissue nuclei that regulate vital reflexes, such as cardiovascular function and respiration. The reticular formation is essential for maintaining wakefulness and in conjunction with the cerebral cortex is referred to as the reticular activating system. Some nuclei within the reticular formation are involved in motor movements.1
TABLE 15-3
DIVISIONS OF THE CENTRAL NERVOUS SYSTEM
PRIMARY VESICLES | SECONDARY VESICLES | ASSOCIATED STRUCTURES |
Forebrain (prosencephalon) | Telencephalon | Cerebral hemispheres |
Cerebral cortex | ||
Rhinencephalon | ||
Basal ganglia | ||
Diencephalon | Epithalamus | |
Thalamus | ||
Hypothalamus | ||
Subthalamus | ||
Midbrain (mesencephalon) | Mesencephalon | Corpora quadrigemina (tectum) |
Cerebral pedunclesTegmentum Red nucleus Substantia nigra Basis pedunculi | ||
Hindbrain (rhombencephalon) | Metencephalon | Cerebellum Pons |
Myelencephalon | Medulla oblongata | |
Spinal cord | Spinal cord | Spinal cord |
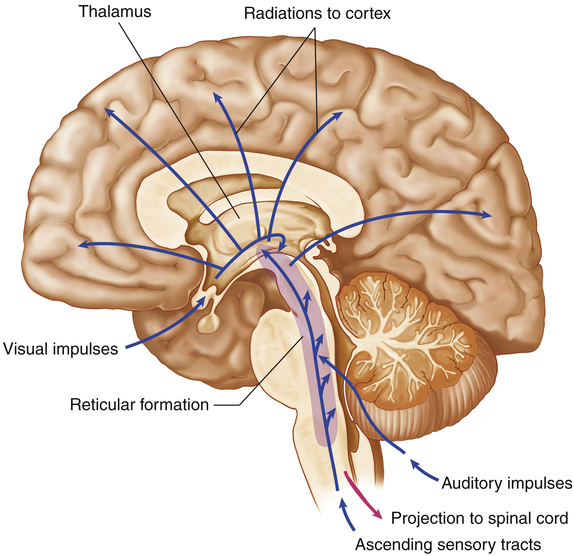
System consists of nuclei in the brainstem reticular formation plus fibers (axons) that conduct to the nuclei from below and fibers that conduct from the nuclei to widespread areas of the cerebral cortex. Functioning of the reticular activating system is essential for consciousness. (From Patton KT, Thibodeau GA: Anatomy & physiology, ed 8, St Louis, 2013, Mosby.)
Many attempts have been made to ascribe function to various regions of the cerebral cortex. (Figure 15-7, B and C, illustrates these regions and identifies some functional areas.) Another basic CNS principle, plasticity, holds that the CNS is capable of change. For example, children with brain damage may experience “relocation” of some functional areas to other parts of the brain. This propensity for plasticity decreases with age, which explains why older individuals tend not to recover from brain injuries as well as younger individuals. This varying balance between specificity and plasticity makes understanding brain functions difficult.
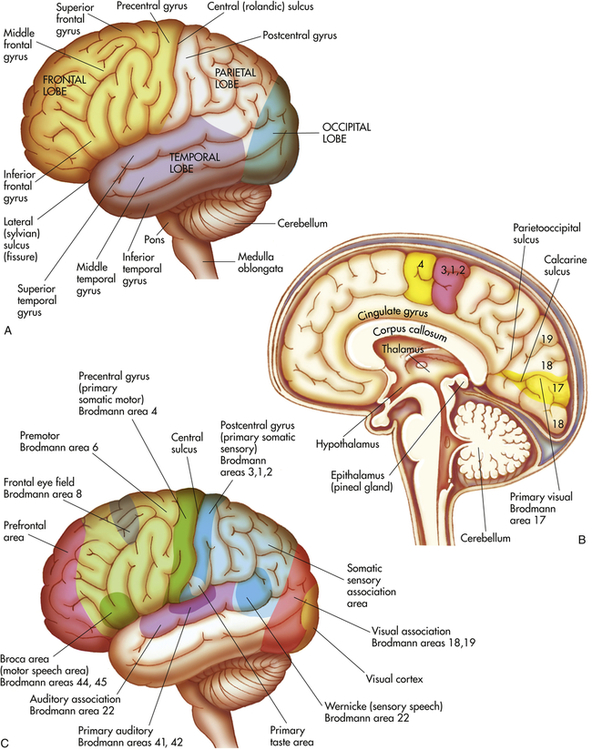
A, Left hemisphere of cerebrum (lateral view). B, Functional areas of the cerebral cortex (midsagittal view). C, Functional areas of the cerebral cortex (lateral view).
Forebrain
Telencephalon
The telencephalon consists of the cerebrum (the largest portion of the brain), which includes the cerebral cortex and basal ganglia. The surface of the cerebrum is characterized by numerous convolutions called gyri (see Figure 15-7, A). The gyri greatly increase the cortical surface area. Grooves between adjacent gyri are called sulci. Deeper grooves are referred to as fissures. The cerebral cortex contains the cell bodies and dendrites of neurons, which often are referred to as gray matter. Gray matter is organized into columns perpendicular to the surface that receive, integrate, store, and transmit information. White matter lies beneath the cerebral cortex and is composed of myelinated nerve fibers, which send neuron “messages” throughout the nervous system and body.
The two cerebral hemispheres are separated by the longitudinal fissure. The surface of each hemisphere is divided into lobes that take their names from the region of the skull under which each of them lies. The posterior margin of the frontal lobe is the central sulcus (fissure of Rolando, central fissure); it borders inferiorly on the lateral sulcus (sylvian fissure, lateral fissure) (see Figure 15-7, A). The prefrontal area is responsible for goal-oriented behavior (i.e., ability to concentrate), short-term or recall memory, and elaboration of thought and inhibition on the limbic (emotional) areas of the CNS. The premotor area (Brodmann area 6) (see Figure 15-7, C) is involved in programming motor movements. This area also contains the neurons that contribute to the basal ganglia system (extrapyramidal system—efferent pathways outside the pyramids of the medulla oblongata). The frontal eye fields (the lower portion of Brodmann area 8), which are involved in controlling eye movements, are located in the middle frontal gyrus.
The primary motor area (Brodmann area 4) is located along the precentral gyrus forming the primary voluntary motor area, which has a somatotopic organization that often is referred to as a homunculus (little man) (Figure 15-8). Electrical stimulation of specific areas of this cortex causes specific muscles of the body to move. The medial part of the cortex in the longitudinal fissure (midline space between the two cerebral hemispheres) affects the lower limb and foot, whereas on the lateral surface, the superior third controls the torso and arm, the middle third the hand, and the lowest third the face and mouth/throat. The axons traveling from the cell bodies in and on either side of this gyrus project fibers (axons) that form the corticospinal tracts (pyramidal system) that descend down the spinal cord. Cerebral impulses control function on the opposite side of the body, a phenomenon called contralateral control (Figure 15-9, A). The Broca speech area (Brodmann areas 44, 45) is rostral to the inferior edge of the premotor area (Brodmann area 6) on the inferior frontal gyrus. It is usually on the left hemisphere and is responsible for the motor aspects of speech. Damage to this area, commonly as a result of a cerebrovascular accident (stroke), results in the inability to form, or difficulty in forming, words (expressive aphasia or dysphasia) (see Chapter 18).
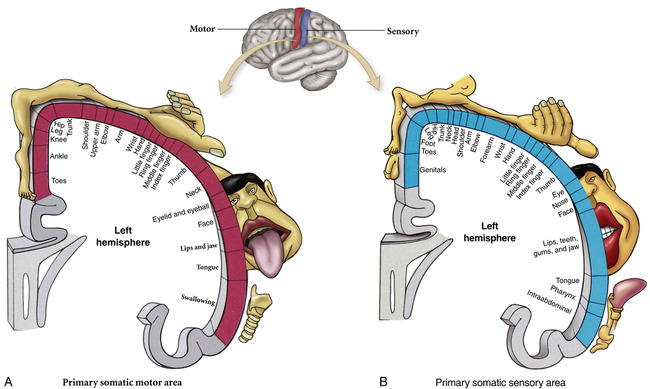
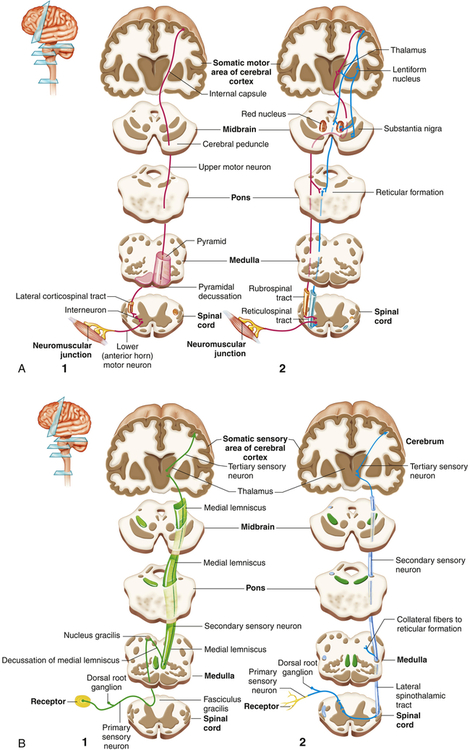
A, Motor: 1, the pyramidal pathway through the lateral corticospinal tract and 2, the extrapyramidal pathways through the rubrospinal and reticulospinal tracts. Note that the pyramidal tracts decussate (cross over) to control the opposite side of the body. B, Sensory: 1, pathways of the medial lemniscal system that conducts information about discriminating touch and kinesthesis and 2, the spinothalamic pathway that conducts information about pain and temperature. (From Patton KT, Thibodeau GA: Anatomy & physiology, ed 8, St Louis, 2013, Mosby.)
The parietal lobe lies within the borders of the central, parietooccipital, and lateral sulci. This lobe contains the major area for somatic sensory input, located primarily along the postcentral gyrus (Brodmann areas 3, 1, 2), which is adjacent to the primary motor area. Communication between the motor and sensory areas (and among other regions in the cortex) is provided by association fibers (i.e., axons from association fibers). Much of this region is involved in sensory association (storage, analysis, and interpretation of stimuli). (Figure 15-8 shows the distribution of functions associated with both the primary motor area and the primary sensory area of the cerebral cortex.)
Another cerebral area, the insula, lies hidden from view deep in the lateral sulcus. Lying directly beneath the longitudinal fissure is a massive white matter pathway called the corpus callosum (commissural fibers). The corpus callosum connects the two cerebral hemispheres and is essential in the coordination of activities between hemispheres, especially specific tasks that may be present in only one hemisphere (see Figures 15-7, C, and 15-15). As a last resort, part or all of the corpus callosum is cut to prevent the spread of epileptic loci (site of seizure activity) through the corpus callosum to the opposite cerebral hemisphere. Epileptic loci often are found in the temporal lobe (see Chapters 17 and 20). This procedure, evolved in the well-known split-brain studies, results initially in temporary aphasia and paralysis.
Functionally, the basal ganglia include, in addition to the corpus striatum, the subthalamic nucleus of the diencephalon and the substantia nigra of the mesencephalon. The basal ganglia plus their interconnections with the thalamus, premotor cortex, red nucleus, reticular formation, and spinal cord are part of the basal ganglia system (extrapyramidal system). The basal ganglia system is believed to exert a fine-tuning effect on motor movements. Parkinson disease and Huntington disease are conditions associated with defects of the basal ganglia (Box 15-2). They are characterized by various involuntary or exaggerated motor movements (see Chapter 17).
The limbic system, first described in 1878 by Broca, is composed of the Papez circuit (amygdala, parahippocampal gyrus, hippocampus, fornix, mamillary body of the hypothalamus, thalamus, and cingulate gyrus), septal area, habenula, nucleus accumbens, and other portions of the hypothalamus, and related autonomic nuclei. It is an extension or modification of the olfactory system (rhinencephalon). Its principal effects are believed to be involved with primitive behavioral responses, visceral reaction to emotion, feeding behaviors, biologic rhythms, and the sense of smell. Expression of affect (emotional and behavioral states) is mediated by extensive connections with the limbic system and prefrontal cortex. The limbic system has as one of its major functions the consolidation of memory through a reverberating circuit (see Chapter 17 and Figure 17-9).
Diencephalon
The diencephalon, surrounded by the cerebrum, is made up of four divisions: epithalamus, thalamus, hypothalamus, and subthalamus (see Table 15-3 and Figure 15-7, B). The epithalamus forms the roof of the third ventricle (a brain cavity) and composes the most superior portion of the diencephalon. It has connections between the limbic system and other parts of the brain. The pineal body (a component of the epithalamus) secretes melatonin, which maintains circadian rhythms and the sleep-wake cycle (see Chapters 16 and 21). The largest component of the diencephalon is the thalamus. It is approximately the size and volume of the thumb from the tip to the first joint. It borders and surrounds the third ventricle, and it is a major integrating center for afferent impulses to the cerebral cortex, except for olfaction. The perception of various sensations occurs at this level but requires cortical processing for interpretation. The thalamus also serves as a relay center for sensory aspects of motor information from the basal ganglia and cerebellum to appropriate cortical motor areas. Cerebral cortical information also projects to the thalamus, creating reverberating circuits.
The hypothalamus forms the base of the diencephalon. Hypothalamic function falls into two major areas: (1) maintenance of a constant internal environment and (2) implementation of behavioral patterns. Integrative centers control function of the ANS, regulation of body temperature, function of the endocrine system, and regulation of emotional expression. (Temperature regulation is discussed in Chapter 16.) The hypothalamus exerts its influence through the endocrine system, as well as through neural pathways (Box 15-3). (For endocrine functions of the hypothalamus and pituitary, see Chapter 21.)
The subthalamus flanks the hypothalamus laterally. The subthalamus contains the subthalamic nucleus, which is part of the basal ganglia system (p. 456).
Midbrain
The midbrain (mesencephalon) (see Table 15-3) is composed of three structures: the corpora quadrigemina, or tectum (composed of the superior and inferior colliculi); the tegmentum (containing the red nucleus and substantia nigra); and the basis pedunculi. (The tegmentum and basis pedunculi are collectively termed the cerebral peduncles―see Figure 15-9, A.)
The superior colliculi are involved with voluntary and involuntary visual motor movements (e.g., the ability of the eyes to track moving objects in the visual field). The inferior colliculi accomplish similar motor activities but involve movements affecting the auditory system (e.g., positioning the head to improve hearing). The inferior colliculus is also a major relay center along the auditory pathway. The red nucleus is a major motor output center that is influenced by the cerebellum. The inferior-most portion of the basal ganglia is the substantia nigra, which synthesizes dopamine, a neurotransmitter and precursor of norepinephrine. Its dysfunction is associated with Parkinson disease (see Chapter 17) and drug addiction (see What’s New? Nucleus Accumbens, Dopamine, and Drug Addiction). The basis pedunculi are made up of efferent fibers of the corticospinal, corticobulbar, and corticopontocerebellar tracts.
Hindbrain
Metencephalon
The major structures of the metencephalon are the cerebellum and the pons. The cerebellum (see Figure 15-7, A and B) is composed of two cerebellar hemispheres covered with small convolutions called folia. Each hemisphere is divided by the primary fissure into two lobes (anterior and posterior) that are connected by a midline structure called the vermis, meaning worm.
cerebral cortex to the contralateral cerebellar hemisphere. The pons is an important center for the control of respiration (i.e., rate and relationship of inspiration to expiration). The nuclei of cranial nerves V through VIII are located in this structure.
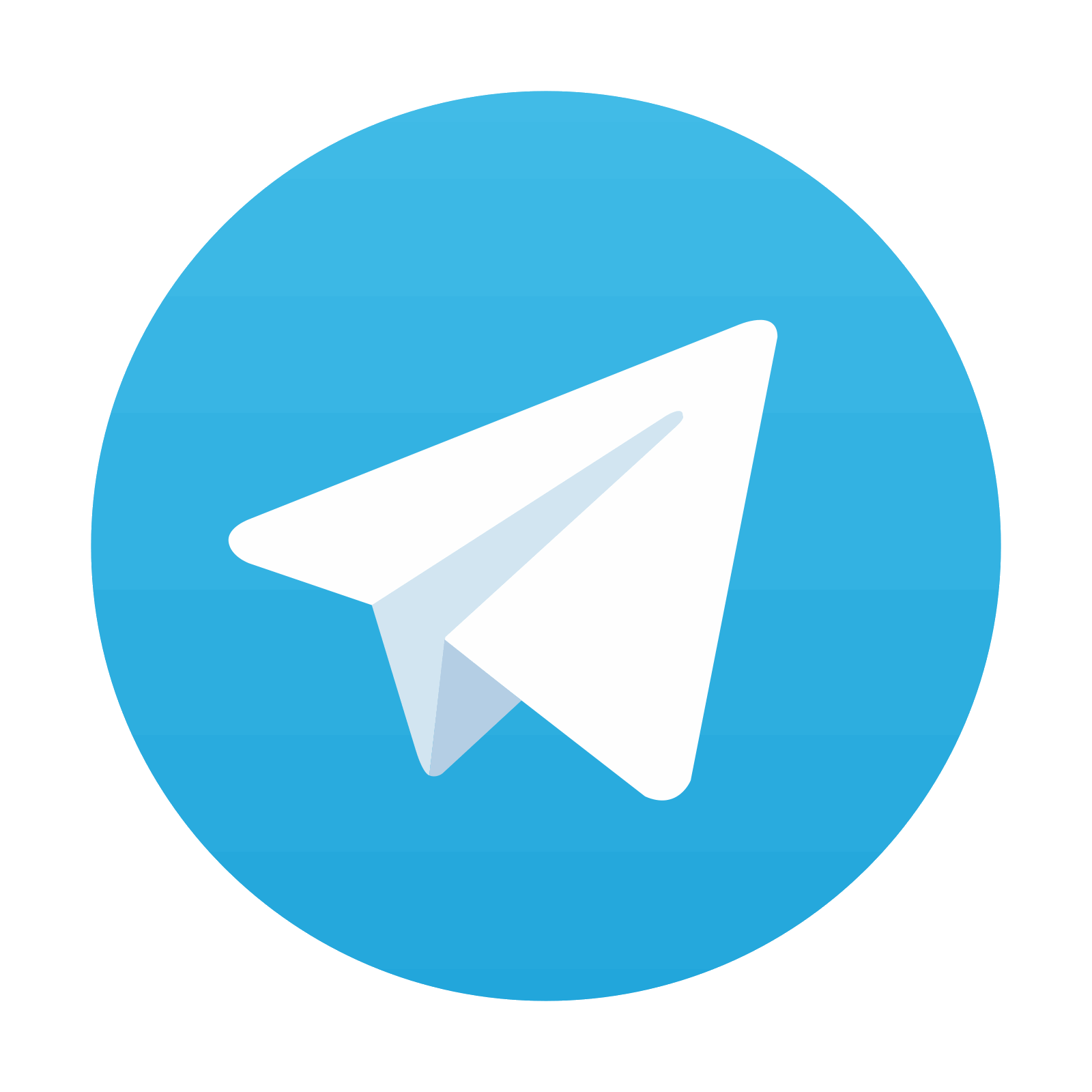
Stay updated, free articles. Join our Telegram channel
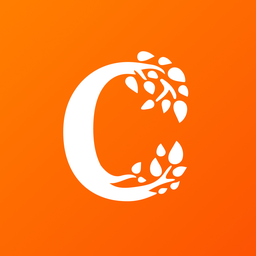
Full access? Get Clinical Tree
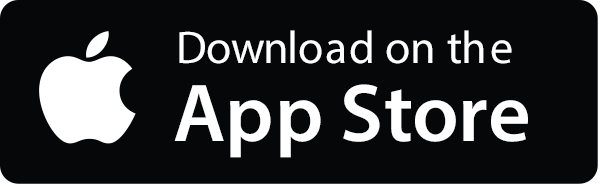
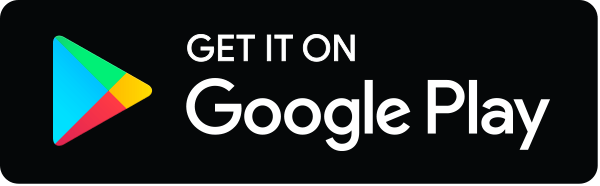