Chapter 22 The timing of the enzyme’s diagnostic window is another important aspect to be considered when these markers are used to evaluate acute injury. According to Noe,52 the diagnostic window for an injury marker is the interval of time following an episode of injury during which plasma concentrations of the marker are increased, thereby demonstrating the occurrence of injury. Marker substances that rapidly enter the circulation (i.e., early indicators) tend to have diagnostic windows that begin soon after onset of the injury. On the contrary, those biomarkers that are slowly released into the circulation and/or are slowly cleared from the circulation (i.e., late indicators) generally have diagnostic windows that begin later and last long after the time of injury. Because of very high concentrations of enzymes within the cells—thousands or even tens of thousands times greater than concentrations in extracellular fluid—and because extremely small amounts of enzyme can be detected by their catalytic activity, increased enzyme activity in the extracellular fluid or plasma is an extremely sensitive indicator of even minor cellular damage, some causes of which are listed in Table 22-1. TABLE 22-1 Causes of Cell Damage or Death Based on the classification of Robbins and colleagues. In: Basic pathology, 3rd edition. Philadelphia: WB Saunders, 1981. The intracellular location of the leaking enzymes affects the rates at which they appear in the circulation. As would be expected, the most sensitive indicators of cell damage are the molecules that are present in the soluble fraction of the cell. Release of structurally bound membrane proteins requires both a leaky cell membrane and a dissociation or degradation, which is a slower process.39 Enzymes associated with subcellular structures, such as mitochondria, are less readily released into the circulation and often indicate irreversible cellular injury. This fact has been used in attempts to distinguish reversible leakage, presumed to reflect damage only to the cell membrane, from necrotic lesions, in which intracellular structures are destroyed. The selection of which enzyme to measure in serum for diagnostic or prognostic purposes depends on a number of factors. An important factor is the distribution of enzymes among the various tissues, shown, for example, for aspartate aminotransferase, alanine aminotransferase, and creatine kinase in Figure 22-2. The main enzymes of established clinical value, together with their tissues of origin and their major clinical applications, are listed in Table 22-2. TABLE 22-2 Distribution of Diagnostically Important Enzymes Enzymes in this category include creatine kinase, aldolase, and glycogen phosphorylase. Optimal pH values for the forward (Cr + ATP → ADP + CrP) and reverse (CrP + ADP → ATP + Cr) reactions are 9.0 and 6.7, respectively. At neutral pH, the formation of ATP is favored; a pH of 9.0 is optimal for the formation of CrP, another high-energy compound. Mg2+ is an obligate activating ion that forms complexes with ATP and ADP. The optimal concentration range for Mg2+ is narrow, and excess Mg2+ is inhibitory. Many metal ions, such as Mn2+, Ca2+, Zn2+, and Cu2+, inhibit enzyme activity, as do iodoacetate and other sulfhydryl-binding reagents. Activity is inhibited by excess ADP and by citrate, fluoride, nitrate, acetate, iodide, bromide, malonate, and L-thyroxine.3 Urate and cystine are potent inhibitors of the enzyme in serum. Even chloride and sulfate ions inhibit activity, and the concentrations of these ions should be kept low in any enzyme assay system based on the CrP + ADP (reverse) reaction. The enzyme in serum is relatively unstable, activity being lost as a result of sulfhydryl group oxidation at the active site of the enzyme. Activity can be partially restored by incubating the enzyme preparation with sulfhydryl compounds, such as N-acetylcysteine, dithiothreitol (Cleland reagent), and glutathione. The current agent of choice is N-acetylcysteine, which has the advantage of being a very soluble substance used at a final concentration of 20 mmol/L in the assay reagent. CK activity is greatest in striated muscle and heart tissue, which contain some 2500 and 550 U/g of protein, respectively. Other tissues, such as the brain, the gastrointestinal tract, and the urinary bladder, contain significantly less activity, and the liver and erythrocytes are essentially devoid of activity (Table 22-3). CK is a dimer composed of two subunits, each with a molecular weight of about 40,000 Da. These subunits (B and M) are the products of loci on chromosomes 14 and 19, respectively. Because the active form of the enzyme is a dimer, only three different pairs of subunits can exist: BB (or CK-1), MB (or CK-2), and MM (or CK-3). The Commission on Biochemical Nomenclature has recommended that isoenzymes be numbered on the basis of their electrophoretic mobility, with the most anodal form receiving the lowest number. Accordingly, the CK isoenzymes are numbered CK-1, CK-2, and CK-3. The distribution of these isoenzymes in the various tissues of humans is shown in Table 22-3. All three of these isoenzyme species are found in the cytosol of the cell or are associated with myofibrillar structures. However, there exists a fourth form that differs from the others both immunologically and by electrophoretic mobility. This isoenzyme (CK-Mt) is located between the inner and outer membranes of mitochondria, and it constitutes, in the heart for example, up to 15% of total CK activity. The gene for CK-Mt is located on chromosome 15. CK activity may also be found in macromolecular form—the so-called macro-CK. Macro-CK is found, often transiently, in the sera of up to 6% of hospitalized patients, but only a minor proportion of these have increased CK activities in serum. It exists in two forms: types 1 and 2. Type 1 is a complex of CK, typically CK-BB, and an immunoglobulin, often IgG, but other complexes have been described, such as CK-MM with IgA. Macro-CK type 1 is not of pathologic significance, but it can be the cause of elevated CK results, resulting in diagnostic confusion and leading to unnecessary further investigation. Prevalence has been estimated as between 0.8 and 2.3%, but this is dependent on the method used and the population studied.17 Macro-CK type 2 is oligomeric CK-Mt, with a reported prevalence of between 0.5 and 2.6%. It is found predominantly in adults who are severely ill with malignancy or liver disease, or in children who have notable tissue distress. The appearance of this isoenzyme in serum is usually associated with a poor prognosis. Macro-CK can interfere with the assay of CK-MB by some immunoinhibition methods. Both M and B subunits have a C-terminal lysine residue, but only the former can be hydrolyzed by the action of carboxypeptidases present in blood. Carboxypeptidases B (EC 3.4.17.2) and N (arginine carboxypeptidase; EC 3.4.17.3) sequentially hydrolyze the lysine residues from CK-MM to produce two CK-MM isoforms: CK-MM2 (one lysine residue removed) and CK-MM1 (both lysine residues removed). Loss of the positively charged lysine produces a more negatively charged CK molecule with greater anodic mobility at electrophoresis. Because CK-MB has only one M subunit, the dimer coded by the M and B genes is named CK-MB2 and the lysine-hydrolyzed dimer is named CK-MB1. The assay of the CK isoforms requires a special technique, such as high-voltage electrophoresis (with gel cooling), high-performance liquid chromatography (HPLC), or immunoassay.61 Elevation of serum CK activity may be the only sign of subclinical neuromuscular disorders.43 In case series, 30 to 44% of asymptomatic subjects with persistent hyperCKemia up to fivefold the upper reference limit (URL) have myopathy. Serum CK activity is greatly elevated in all types of muscular dystrophy. In progressive muscular dystrophy (particularly Duchenne sex-linked muscular dystrophy), enzyme activity in serum is highest in infancy and childhood (7 to 10 years of age) and may be increased long before the disease is clinically apparent. Serum CK activity characteristically falls as patients get older and as the mass of functioning muscle diminishes with progression of the disease. About 50 to 80% of asymptomatic female carriers of Duchenne dystrophy show threefold to sixfold increases in CK activity. High values of CK are noted in viral myositis, polymyositis, and similar muscle diseases. However, in neurogenic muscle diseases, such as myasthenia gravis, multiple sclerosis, poliomyelitis, and parkinsonism, serum enzyme activity is not increased. Very high activity is also encountered in malignant hyperthermia, an inherited life-threatening condition characterized by high fever and brought on by administration of inhalation anesthesia (usually halothane) to the affected individual. In acute rhabdomyolysis due to crush injury, with severe muscle destruction, serum CK activities exceeding 200 times the URL may be found. If the CK remains below 5000 U/L (about 30 times the URL) during the first 3 days after the insult, the probability of developing acute renal failure appears to be low.5 Serum CK can also be increased by other direct trauma to muscle, including intramuscular injection and surgical intervention. Finally, a number of drugs when given at pharmacologic doses can increase serum CK activities. The drugs principally responsible are statins, fibrates, antiretrovirals, and angiotensin II receptor antagonists. Varying degrees of myopathy may occur with statin use, ranging from mild myalgic syndrome alone to rhabdomyolysis (0.02%).86 Changes in serum CK and its MB isoenzyme following acute myocardial infarction have been the mainstay of diagnosis for many years.14 However, it is now more advantageous to use more cardiac-specific nonenzymatic markers, such as cardiac troponin I or T. CK-MB determination can still be used with some success to estimate the extent of myocardial necrosis to assist with assessment of infarct prognosis. When peak CK-MB is compared with estimates of infarct size, good correlations can be obtained (Table 22-4). A problem with using CK-MB for this purpose is the requirement for frequent sampling to ensure that peak CK-MB values are correctly identified. TABLE 22-4 Decision Limits of CK-MB Mass Peak for Infarct Size Definition CK catalyzes the conversion of CrP to Cr with concomitant phosphorylation of ADP to ATP. The ATP produced is measured by hexokinase (HK)/glucose-6-phosphate dehydrogenase (G6PD) coupled reactions that ultimately convert NADP+ to NADPH, which is monitored spectrophotometrically at 340 nm. Szasz and colleagues optimized the assay by adding N-acetylcysteine to activate CK, EDTA to bind Ca2+ and to increase the stability of the reaction mixture, and adenosine pentaphosphate (Ap5A) in addition to AMP to inhibit adenylate kinase (AK).85 A reference method based on this previous experience was developed by the International Federation of Clinical Chemistry and Laboratory Medicine (IFCC) for the measurement of CK at 37 °C.76 Serum CK activity is subject to a number of physiologic variations. It is influenced by sex, age, race, muscle mass, and physical activity. The distributions of CK activity are notably skewed toward higher values in reference populations. Men have higher values than women, and blacks have higher values than nonblacks. In white subjects, the reference interval was found to be 46 to 171 U/L for males and 34 to 145 U/L for females, when measured with an assay traceable to the IFCC 37 °C reference procedure.81 Newborns generally have higher CK activity resulting from skeletal muscle trauma during birth. Serum CK in infants decreases to the adult reference interval by 6 to 10 weeks. CK activity in the serum of healthy people is due almost exclusively to CK-MM activity (although small amounts of CK-MB may be present) and is the result of physiologic turnover of muscle tissue. Exercise and muscle trauma can increase serum CK.7 Sustained exercise, such as that performed by well-trained long-distance runners, increases the CK-MB content of skeletal muscle, which may produce abnormal serum CK-MB concentrations. Electrophoretic methods are useful for separation of all CK isoenzymes. The isoenzyme bands are visualized by incubating the support (e.g., agarose, cellulose acetate) with a concentrated CK assay mixture using the reverse reaction. NADPH formed in this reaction is then detected by observing the bluish-white fluorescence after excitation by long-wave (360 nm) ultraviolet light. NADPH may be quantified by fluorescent densitometry, which is capable of detecting bands of 2 to 5 U/L. The mobility of CK isoenzymes at pH 8.6 toward the anode is BB > MB > MM, with the MM remaining cathodic to the point of application. The discriminating power of electrophoresis also allows the detection of abnormal bands (Figure 22-3). Disadvantages of electrophoresis include that the turnaround time is relatively long, the procedure is highly labor intensive and is not adaptable to clinical chemistry analyzers, and interpretative skills are required. Currently, the most common approach is to measure concentrations of the CK-MB protein (“mass”) by using immunoassays with monoclonal antibodies.58 Measurements use the “sandwich” technique, in which one antibody specifically recognizes only the MB dimer. The sandwich technique ensures that only CK-MB is estimated because neither CK-MM nor CK-BB reacts with both antibodies. Mass assays are more sensitive than activity-based methods with a limit of detection for CK-MB usually less than 1 µg/L. Other advantages include sample stability, noninterference with hemolysis, anticoagulants or other catalytic activity inhibitors, full automation, and fast turnaround time. With CM-MB mass assays, the upper reference limit for males is 5.0 µg/L, with values for females being less than male values, although many laboratories use a single reference limit (male). Serum ALD determinations have been of some clinical interest in primary diseases of skeletal muscle. Some researchers believe that increased ALD activity is useful in distinguishing neuromuscular atrophies from myopathies in combination with the CK/AST ratio.27 In general, however, measurement of ALD activity in the serum of subjects with suspected muscle disease does not add information to that available more readily from measurement of other enzymes, especially CK.22 At the time of this book’s writing, ALD measurement has largely been discontinued within clinical laboratories and is not routinely available. The reference interval for the activity of ALD in adults is 2.5 to 10 U/L, measured at 37 °C. However, a definite sex difference has been noted, with men having higher values. Serum ALD activity in the neonate is fourfold that seen in adult, and in children is twice that in the adult. Adult values are attained by the time the child reaches puberty.91 In preliminary studies, GP-BB was significantly more sensitive than CK-MB and myoglobin for the diagnosis of acute myocardial infarction within the first 4 hours after onset of chest pain.2 However, GP-BB is not a heart-specific protein, and its specificity as a marker for myocardial damage is limited. ALT catalyzes the analogous reaction as follows: Transaminases are widely distributed throughout the body. AST is found primarily in the heart, liver, skeletal muscle, and kidney, whereas ALT is found primarily in the liver and kidney, with lesser amounts in heart and skeletal muscle (Table 22-5). ALT is exclusively cytoplasmic; both mitochondrial and cytoplasmic forms of AST are found in cells. These are genetically distinct isoenzymes with a dimeric structure composed of two identical polypeptide subunits of about 400 amino acid residues. TABLE 22-5 Transaminase Activities in Human Tissues, Relative to Serum as Unity From King J. Practical clinical enzymology. London: D Van Nostrand Co Ltd, 1965. Persistence of increased ALT for longer than 6 months after an episode of acute hepatitis is used to diagnose chronic hepatitis. Most patients with chronic hepatitis have maximum ALT less than seven times the upper reference limit. ALT may be persistently normal in 15 to 50% of patients with chronic hepatitis C, but the likelihood of continuously normal ALT decreases with an increasing number of measurements. In patients with acute hepatitis C, ALT should be measured periodically over the next 1 to 2 years to determine if it becomes and stays normal.15 The picture in toxic hepatitis is different from that in infectious hepatitis. In acetaminophen-induced hepatic injury, the transaminase peak is more than 85 times the upper reference limit in 90% of cases—a value rarely seen with acute viral hepatitis. Furthermore, AST and ALT activities typically peak early and fall rapidly.15 Twofold to fivefold elevations of both enzymes occur in patients with primary or metastatic carcinoma of the liver, with AST usually being higher than ALT, but activities are often normal in the early stages of malignant infiltration of the liver. Slight or moderate elevations of AST and ALT activities have been observed after administration of various medications, such as nonsteroidal anti-inflammatory drugs, antibiotics, antiepileptic drugs, statins, or opiates. Over-the-counter medications and herbal preparations are also implicated. In patients with increased transaminases, negative viral markers, and a negative history for drugs or alcohol ingestion, the work-up should include less common causes of chronic hepatic injury (e.g., hemochromatosis, Wilson’s disease, autoimmune hepatitis, primary biliary cirrhosis, sclerosing cholangitis, celiac disease, α1-antitrypsin deficiency).67 Although serum activities of both AST and ALT become elevated whenever disease processes affect liver cell integrity, ALT is the more liver-specific enzyme. Serum elevations of ALT activity are rarely observed in conditions other than parenchymal liver disease.34 Moreover, elevations of ALT activity persist longer than do those of AST activity. Thus the incremental benefit of determination of AST, in addition to ALT, may be limited. Generally, mitochondrial AST (m-AST) activity in serum shows a marked increase in patients with extensive liver cell degeneration and necrosis. Of particular interest is the usefulness of the ratio between m-AST and total AST activities for diagnosing alcoholic hepatitis. The ratio seems to identify the liver cell “necrotic type” condition (i.e., slight enzyme increase concomitant with relatively high activities of mitochondrial enzymes) typical of alcoholic hepatitis.56 Several authors have described AST linked to immunoglobulins, or macro-AST. Typical findings include a persistent increase in serum AST activity in an asymptomatic subject, with absence of any demonstrable pathology in organs rich in AST. Increased AST activity might reflect decreased clearance of the abnormal complex from plasma. Macro-AST has no known clinical relevance. However, identification is important to avoid unnecessary diagnostic procedures in these subjects. Laboratory procedures for the demonstration of macro-AST include electrophoresis with specific enzyme stain (atypical origin band) and differential precipitation with polyethylene glycol (PEG) 6000 (see “Amylase” section later in this chapter). Because of the large numbers of AST and ALT activity measurements performed daily in clinical laboratories throughout the world, standardization of transaminase measurements is a priority need for patient care. As discussed in Chapter 8, the reference system approach, based on the concepts of metrologic traceability and the hierarchy of analytical measurement procedures, gives clinical laboratories and the medical community universal means of creating and ensuring the comparability of results. In this system, the IFCC reference measurement procedure forms the highest metrologic level and thereby constitutes the definition of the respective measurable enzyme quantity.30 Primary IFCC procedures for the measurement of catalytic activity concentrations of AST and ALT at 37 °C have been published.79,80 Values assigned to the manufacturer’s product calibrators and measurement results of lower metrologic levels, including those used in daily routine practice, should be traceable to these top-level reference measurement procedures, thus improving the accuracy and comparability of transaminase results. It should be remembered that the concept of the reference system is valid only if the reference procedure and corresponding routine procedures have identical, or at least very similar, specificities for the measured enzyme. Thus it will not be possible to calibrate procedures for aminotransferases that do not incorporate P-5′-P using a procedure that does, such as the IFCC reference procedure, because the ratio of pre-formed holoenzyme to apoenzyme differs among specimens. AST isoenzymes can be separated into anionic (cytoplasmic AST) and cationic bands (m-AST) by electrophoresis. However, the low concentration of m-AST in normal sera is usually below the limit of detection of this method. Immunoprecipitation assays using antibodies directed against both mitochondrial and cytosolic isoenzymes allow instead measurement of low concentrations of the m-AST isoenzyme present in serum. A homogeneous inhibition assay using proteinase K (EC 3.4.21.14) for selective proteolysis of cytosolic AST has been described and made amenable to automation, permitting m-AST to be measured with convenience that approaches that of the total AST assay.63 Continuous-monitoring methods have been developed for determination of GLD using both forward and reverse reactions. The equilibrium favors the formation of glutamate, and higher reaction rates are observed when 2-oxoglutarate is used as a substrate. Serum is added to a solution of NADH, an ammonium salt, and ADP in buffer at pH 7.5, and the reaction is initiated by the addition of the substrate, 2-oxoglutarate. The rate of decrease in absorbance at 340 nm is measured. The German Society for Clinical Chemistry has published optimum reaction conditions for 37 °C.13 Oxamate is incorporated into the reaction mixture because this acid inhibits LD activity, avoiding the critical consumption of NADH by this enzyme in serum. GLD activity in serum is stable at 4 °C for 48 hours and at −20 °C for several weeks. ALP exists in multiple forms, some of which are true isoenzymes, encoded at separate genetic loci (Figure 22-4).47 Bone, liver, and kidney ALP forms share a common primary structure coded for by the same genetic locus, but they differ in carbohydrate content.45
Serum Enzymes
Diagnostic Enzymology
Factors Affecting Enzyme Concentrations in Plasma or Serum35,36
Leakage of Enzymes from Cells
Efflux of Enzymes from Damaged Cells
Selection of Enzyme Tests
Enzyme
Principal Sources of Enzyme in Blood
Principal Clinical Applications
Alanine aminotransferase
Liver
Hepatic parenchymal disease
Alkaline phosphatase
Liver, bone, intestinal mucosa, placenta
Hepatobiliary disease, bone disease
Amylase
Salivary glands, pancreas
Pancreatic disease
Aspartate aminotransferase
Heart, liver, skeletal muscle, erythrocytes
Hepatic parenchymal disease
Creatine kinase
Skeletal muscle, heart
Muscle disease, myocardial infarction
γ-Glutamyltransferase
Liver, pancreas, kidney
Hepatobiliary disease
Lactate dehydrogenase
Heart, erythrocytes, lymph nodes, skeletal muscle, liver
Hemolytic and megaloblastic anemias, leukemia and lymphomas, oncology
Lipase
Pancreas
Pancreatic disease
5′-Nucleotidase
Liver
Hepatobiliary disease
Muscle Enzymes
Creatine Kinase
Clinical Significance
Microscopic myocardial infarction (focal necrosis)
<10 µg/L
Small myocardial infarction (<10% left ventricle)
10-60 µg/L
Medium myocardial infarction (10-30% left ventricle)
60-225 µg/L
Large myocardial infarction (>30% left ventricle)
>225 µg/L
Methods for Determination of Creatine Kinase Activity
Reference Intervals
Methods for Separation and Quantification of Creatine Kinase Isoenzymes
Aldolase
Clinical Significance
Reference Intervals
Glycogen Phosphorylase
Clinical Significance
Liver Enzymes
Aminotransferases
AST
ALT
Heart
7800
450
Liver
7100
2850
Skeletal muscle
5000
300
Kidneys
4500
1200
Pancreas
1400
130
Spleen
700
80
Lungs
500
45
Erythrocytes
15
7
Serum
1
1
Clinical Significance
Methods for Measurement of Transaminase Activity
Methods for Separation and Quantification of AST Isoenzymes
Glutamate Dehydrogenase
Methods for Determination of Glutamate Dehydrogenase Activity
Alkaline Phosphatase
Stay updated, free articles. Join our Telegram channel
Full access? Get Clinical Tree
Serum Enzymes
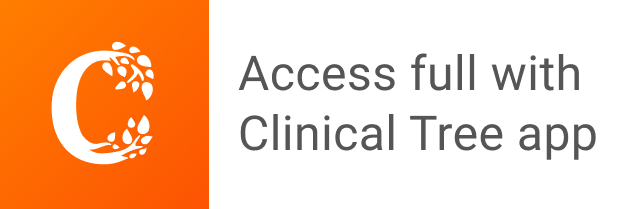