INTRODUCTION
Proteins have the most dynamic and diverse roles of any macromolecules in the body, catalyzing biochemical reactions, constituting receptors and channels in membranes, providing intracellular and extracellular scaffolding support, and transporting molecules within a cell or from one organ to another. According to current estimates, there are approximately 19,000–20,000 protein-coding genes in the human genome, and with alternative splicing of genes and post-translational modification of proteins (e.g., by cleavage, phosphorylation, acylation and glycosylation), the number of functionally distinct proteins is likely to be greater than 100,000. Viewed from the perspective of disease mechanisms, these estimates pose an immense challenge to modern medicine, as disease may result when any one of these proteins contains mutations or other abnormalities or is present in an abnormally high or low concentration. Viewed from the perspective of therapeutics, however, these estimates represent a tremendous opportunity in terms of harnessing protein therapeutics to alleviate disease. At present, more than 206 different proteins or peptides are approved for clinical use by the US Food and Drug Administration (FDA), and many more are in development.
Protein therapeutics have several advantages over small-molecule drugs. First, proteins often serve a highly specific and complex set of functions that cannot be mimicked by simple chemical compounds. Second, because the action of proteins is highly specific, there is often less potential for protein therapeutics to interfere with normal biological processes and cause adverse effects. Third, because the body naturally produces many of the proteins that are used as therapeutics, these agents are often well tolerated and are less likely to elicit immune responses. Fourth, for diseases in which a gene is mutated or deleted, protein therapeutics can provide effective replacement treatment without the need for gene therapy, which is not currently available for most genetic disorders. Fifth, the clinical development and FDA approval time of protein therapeutics may be faster than that of small-molecule drugs. A study published in 2003 showed that the average clinical development and approval time was more than 1 year faster for 33 protein therapeutics approved between 1980 and 2002 than for 294 small-molecule drugs approved during the same time period. Sixth, because proteins are unique in form and function, companies are able to obtain far-reaching patent protection for protein therapeutics. Seventh, many protein therapeutics address unmet needs for rare diseases and are eligible for orphan drug designation, which can provide priority review by the FDA and extended patent protection terms. Last, the total sales of protein therapeutics are continuing to trend upward. In the year 2000, no protein therapeutics were represented in the top 20 drugs sold in the United States. In 2013, protein therapeutics comprised four of the top ten pharmaceuticals by sales in the United States and seven of the top ten pharmaceuticals by sales worldwide. The last four advantages make proteins attractive from a financial perspective compared with small-molecule drugs.
A relatively small number of protein therapeutics are purified from their native sources, such as pancreatic enzymes from hog and pig pancreas and α-1-proteinase inhibitor from pooled human plasma. Instead, most therapeutic proteins are now produced by recombinant DNA technology and purified from a wide range of organisms. Production systems for recombinant proteins include bacteria, yeast, insect cells, mammalian cells, and transgenic animals and plants. The system of choice can be dictated by the cost of production or the modifications of the protein (e.g., glycosylation, phosphorylation, or proteolytic cleavage) that are required for biological activity. For example, bacteria do not perform glycosylation reactions, and each of the other biological systems listed above produces a different type or pattern of glycosylation. Protein glycosylation patterns can have a dramatic effect on the activity, half-life, and immunogenicity of the recombinant protein in the body. For example, the half-life of native erythropoietin, a growth factor important in erythrocyte production, can be lengthened by increasing the glycosylation of the protein. Darbepoetin-α is an erythropoietin analogue that is engineered to contain two additional amino acids that are substrates for N-linked glycosylation reactions. When expressed in Chinese hamster ovary cells, the analogue is synthesized with five rather than three N-linked carbohydrate chains; this modification causes the half-life of darbepoetin to be three times longer than that of erythropoietin.
Perhaps the best example of trends in the production and use of protein therapeutics is provided by the history of insulin in the treatment of type 1 and type 2 diabetes mellitus. Untreated, type 1 diabetes is a disease that leads to severe wasting and death due to lack of the protein hormone insulin, which signals cells to perform numerous functions related to glucose homeostasis and intermediary metabolism. In 1922, insulin was first purified from bovine and porcine pancreas and used as a life-saving daily injection for patients with type 1 diabetes. At least three problems hindered the widespread use of this protein therapy: first, the availability of animal pancreases for purification of insulin; second, the cost of insulin purification from animal pancreas; and third, the immunological reaction of some patients to animal insulin. These problems were addressed by isolating the human insulin gene and engineering Escherichia coli to express human insulin by using recombinant DNA technology. By growing vast quantities of these bacteria, large-scale production of human insulin was achieved. The resulting insulin was abundant, inexpensive, of low immunogenicity, and free from other animal pancreatic substances. Recombinant insulin, approved by the FDA in 1982, was the first commercially available recombinant protein therapeutic and has been the major therapy for type 1 diabetes (and a major therapy for type 2 diabetes) ever since.
Recombinantly produced proteins can have several further benefits compared with nonrecombinant proteins. First, transcription and translation of an exact human gene can lead to a higher specific activity of the protein and a decreased chance of immunological rejection. Second, recombinant proteins are often produced more efficiently and inexpensively and in potentially limitless quantity. One striking example is found in the protein-based therapy for Gaucher’s disease, a chronic congenital disorder of lipid metabolism caused by a deficiency of the enzyme β-glucocerebrosidase (also known as glucosylceramidase) that is characterized by an enlarged liver and spleen, increased skin pigmentation, and painful bone lesions. At first, β-glucocerebrosidase purified from human placenta was used to treat this disease, but this requires purification of protein from 50,000 placentas per patient per year, which clearly places a practical limit on the amount of purified protein available. A recombinant form of β-glucocerebrosidase was subsequently developed and introduced, which is not only available in sufficient quantities to treat many more patients with the disease but also eliminates the risk of transmissible (e.g., viral or prion) diseases associated with purifying the protein from human placentas. This also illustrates a third benefit of recombinant proteins over nonrecombinant proteins—the reduction of exposure to animal or human diseases.
A fourth advantage is that recombinant technology allows the modification of a protein or the selection of a particular gene variant to improve function or specificity. Again, recombinant β-glucocerebrosidase provides an interesting example. When this protein is made recombinantly, a change of amino acid arginine-495 to histidine allows the addition of mannose residues to the protein. The mannose is recognized by endocytic carbohydrate receptors on macrophages and many other cell types, allowing the enzyme to enter these cells more efficiently and to cleave the intracellular lipid that has accumulated in pathological amounts, which results in an improved therapeutic outcome. Last, recombinant technology allows the engineering and production of proteins that provide a novel function or activity, as discussed below.
The more than 30 years since the approval of recombinant insulin by the FDA have seen a remarkable expansion in the number of therapeutic applications of proteins. More than 206 unique proteins (over 160 of which are produced recombinantly) are currently approved for clinical use by the FDA, and many more are in development.
MR is a 55-year-old traveling salesman who presents to the emergency department of a small rural hospital with left-sided chest pain and light-headedness. The pain started suddenly 1 hour ago when he was carrying a large box. At first, MR felt as if he was going to pass out, but the pain and light-headedness improved at rest and eventually resolved after 20 minutes. MR denies any other symptoms, and he has no history of medical problems. He takes no medications, he is not a smoker, and his father died unexpectedly in a car accident at age 53. On physical exam, MR is afebrile with heart rate 100 beats/min, blood pressure 150/90 mm Hg, and respiratory rate 16 breaths/min. His pulse oximeter displays 96% with oxygen flowing at 2 liters per minute by nasal cannula. He appears to be comfortable, and the remainder of his physical exam is notable only for an S4 heart sound. His ECG demonstrates sinus tachycardia with no ST segment elevation. His chest x-ray is normal. His STAT chemistry panel shows normal sodium, potassium, chloride, bicarbonate, blood urea nitrogen (BUN), and creatinine levels. Cardiac biomarkers and coagulation studies are pending. MR is given aspirin, metoprolol, and sublingual nitroglycerin upon his arrival in the emergency department.
While waiting in the emergency department, MR’s troponin T level returns at 1.34 ng/mL (normal, 0–0.1 ng/mL), he again develops chest pain, and a repeat EKG shows 2-mm ST segment depression in leads V1–V3. At this time, he is also given heparin, abciximab, and clopidogrel, and his chest pain resolves. He is admitted to the hospital and his clinical course is stable overnight.
The next day, however, MR develops crushing substernal chest pain and diaphoresis, and his ECG shows 4-mm ST segment elevation in leads V2–V4. Because cardiac catheterization is not available at the regional cardiac center for at least 4 hours, MR is given tenecteplase in the coronary care unit, and his aspirin, metoprolol, nitroglycerin, heparin, and clopidogrel are continued. He stabilizes on this regimen.
After an otherwise uneventful 5-day hospitalization, MR is transferred to the regional cardiac center for catheterization, with a diagnosis of unstable angina that evolved into an ST elevation myocardial infarction. Outpatient plans include cardiac rehabilitation and treatment with aspirin, metoprolol, enalapril, spironolactone, and sublingual nitroglycerin as needed.
Questions
1. By what mechanism does tenecteplase act?
2. How does the action of tenecteplase differ from that of heparin?
3. By what mechanism does abciximab act?
4. How could abciximab augment the function of clopidogrel and aspirin in this case?
An appreciation of the many therapeutic uses of proteins may be facilitated by categorizing such therapies according to their mechanism of action. In this chapter, we summarize currently approved protein therapeutics using a classification system that is based on their pharmacologic action (Box 54-1). Examples of protein therapeutics in each category and clinical conditions in which they are used are discussed in the text, and a listing of FDA-approved protein therapies and their functions and clinical uses is presented in Tables 54-1 through 54-5. Examples of protein-based vaccines and diagnostics, which also highlight the growing importance of proteins in medicine, are provided in Tables 54-6 and 54-7.
BOX 54-1 Functional Classification of Protein Therapeutics |
Protein therapeutics in the tables are organized by function and therapeutic application. The numbers of therapeutics per group reflect the relative difficulty associated with drug development across the various classes of protein therapeutics. Every effort has been made to include in these tables all US Food and Drug Administration (FDA)-approved Group I and Group II protein-based therapies. Groups III and IV present selected examples that highlight the use of proteins in vaccines and diagnostic agents. Group I: Enzymes and Regulatory Proteins
Endocrine and metabolic disorders with defined molecular etiologies dominate Group Ia. As more diseases are linked to deficiencies of specific proteins, this class will continue to grow. Group Ib is dominated by therapies that augment hematological and endocrine pathways and immune responses. The many interferon and growth factor therapies in Group Ib effectively treat disease even when their precise pharmacologic mechanism of action is unknown. Group Ic demonstrates the rational use of naturally occurring proteins to modify the pathophysiology of human diseases. The future growth of this class depends on understanding protein function in human physiology as well as protein function in other organisms. Group II: Targeted Proteins
Group IIa therapeutics use their special targeting activity to interfere with molecules or organisms by binding specifically to them and blocking their function, targeting them for destruction, or stimulating a signaling pathway. This group has grown as monoclonal antibody technology has matured and will expand further as signaling pathways and etiologies of disease are more clearly identified. Group IIb therapeutics deliver other compounds or proteins to a specific site. This class has great potential to grow, as demonstrated by the breadth of the specifically targeted Group IIa therapies. Group III: Protein Vaccines
Although this is currently a small class of therapies, there is great potential for the production of recombinant vaccines that provide broad protection against infectious agents. Similarly, individualized vaccines against cancers are likely to be in great demand. Selected examples of the more than 80 FDA-approved vaccines in Table 54-6 highlight the use of recombinant protein technology in vaccine production. Many of the FDA-approved vaccines protect against multiple infectious agents and include synthetic, recombinant, and purified protein components. A complete list of FDA-approved vaccines may be found at http://www.fda.gov/BiologicsBloodVaccines/Vaccines/ApprovedProducts. Group IV: Protein Diagnostics Protein diagnostics, of which selected examples are shown in Table 54-7, are a class that powerfully affects clinical decision making. These diagnostics use technology and therapeutics developed in other classes to answer clinical questions. This table presents primarily in vivo protein diagnostics, but in vitro protein diagnostics are also critical to medical decision making and are too numerous to address comprehensively here. |
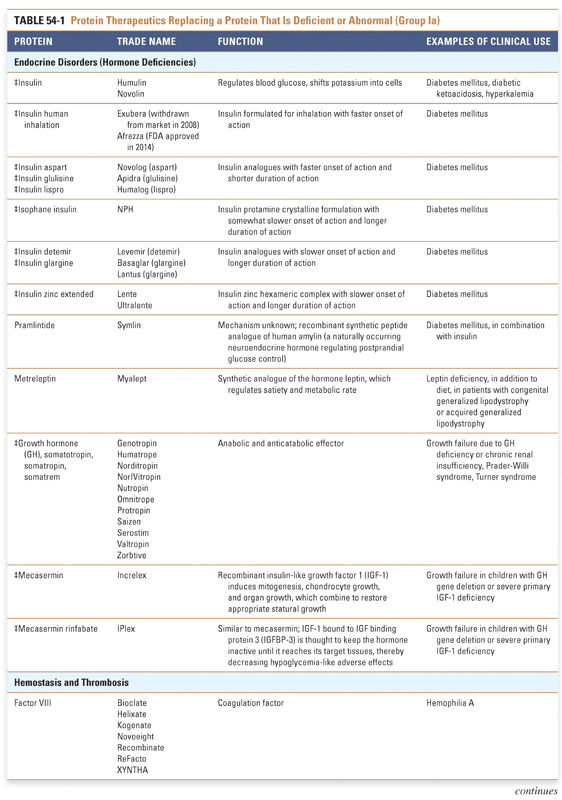
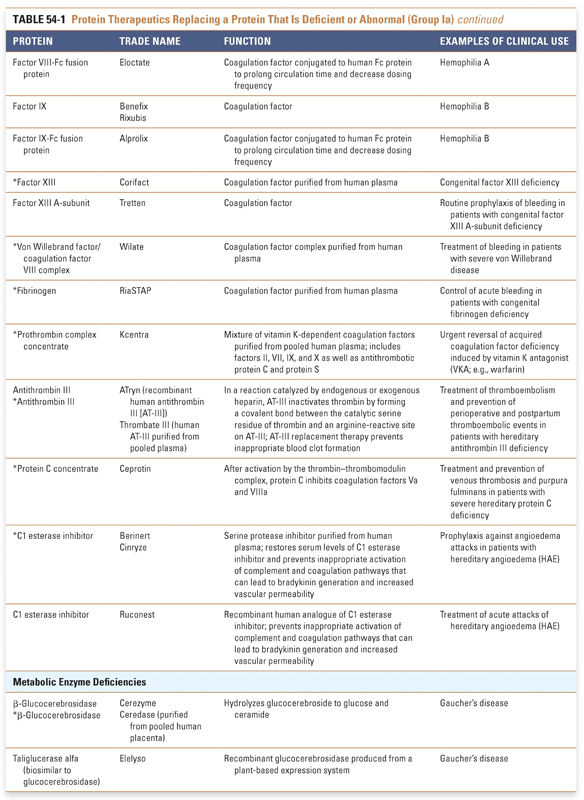
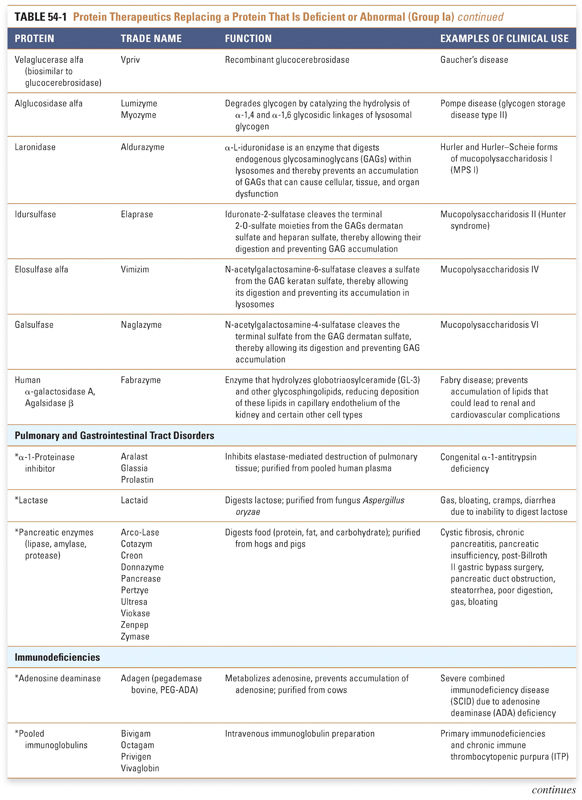
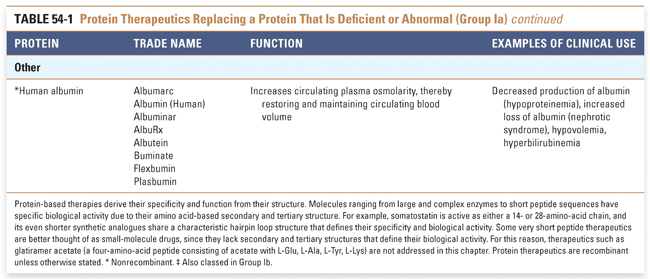
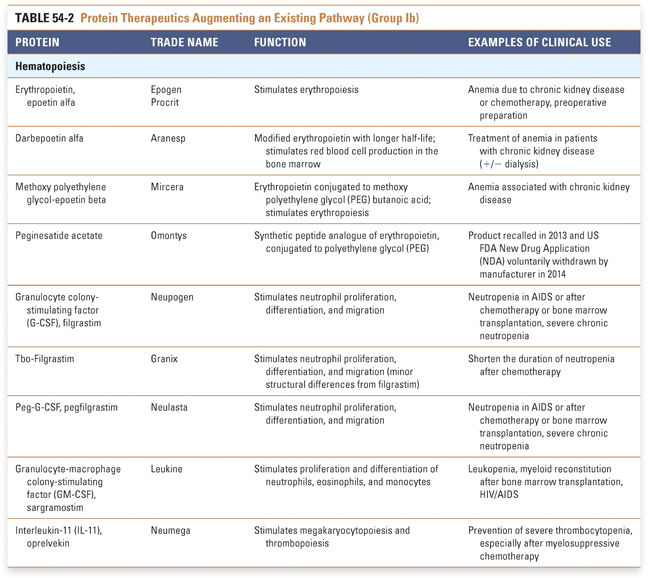
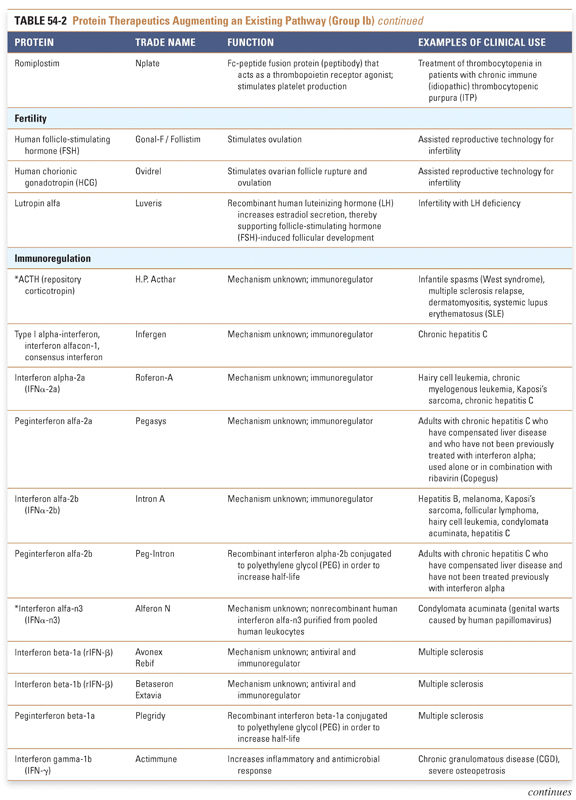
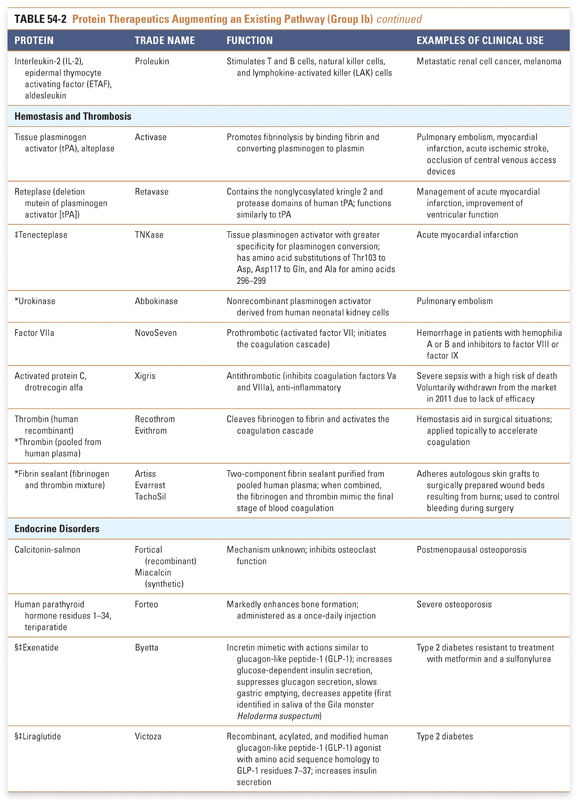
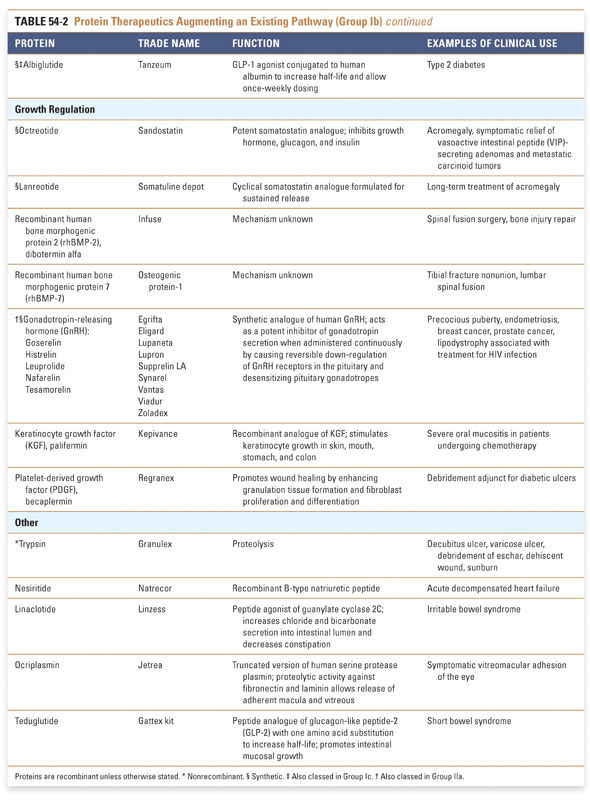
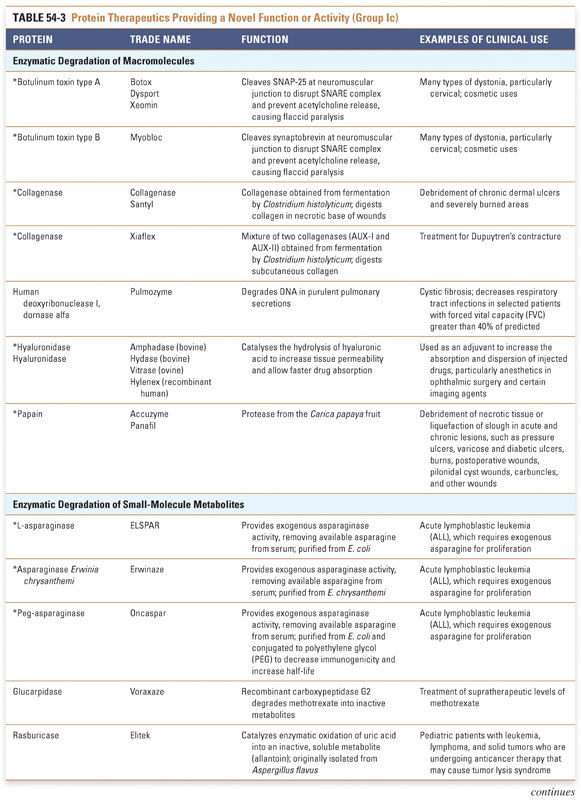
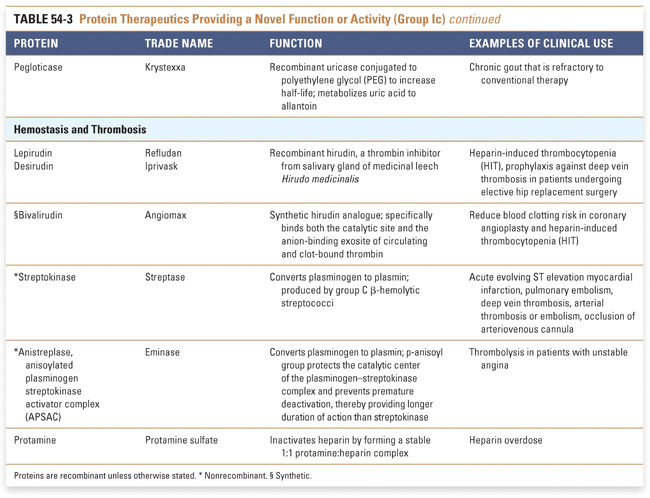
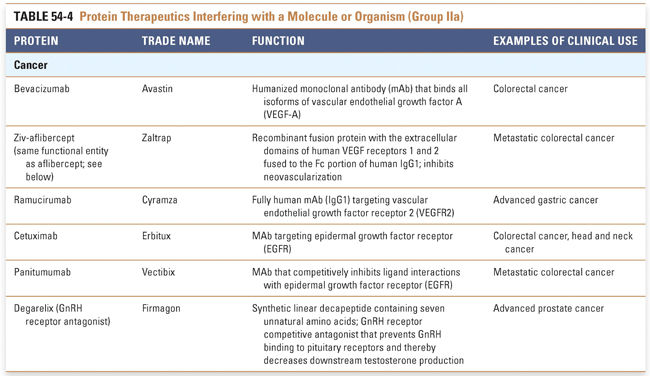
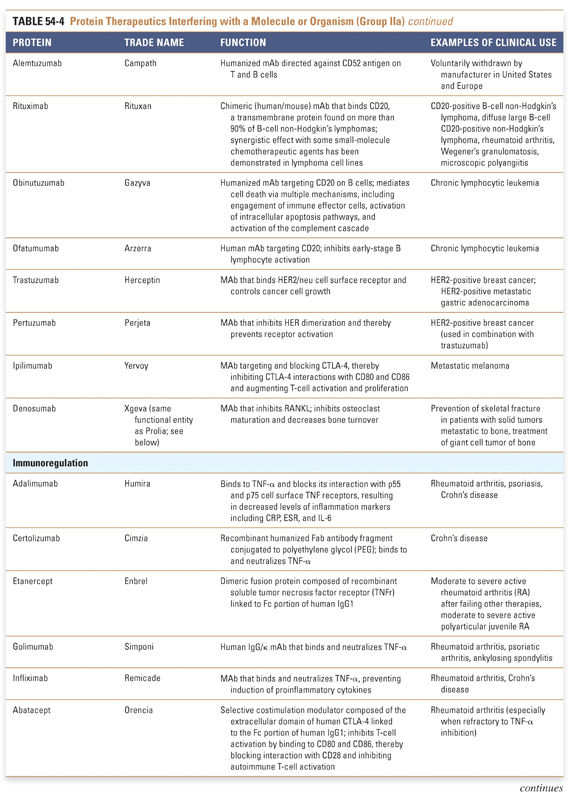
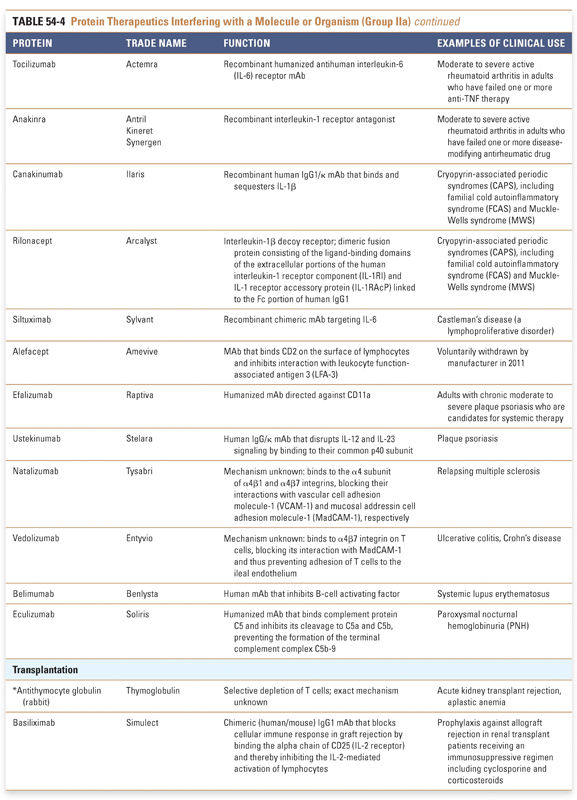
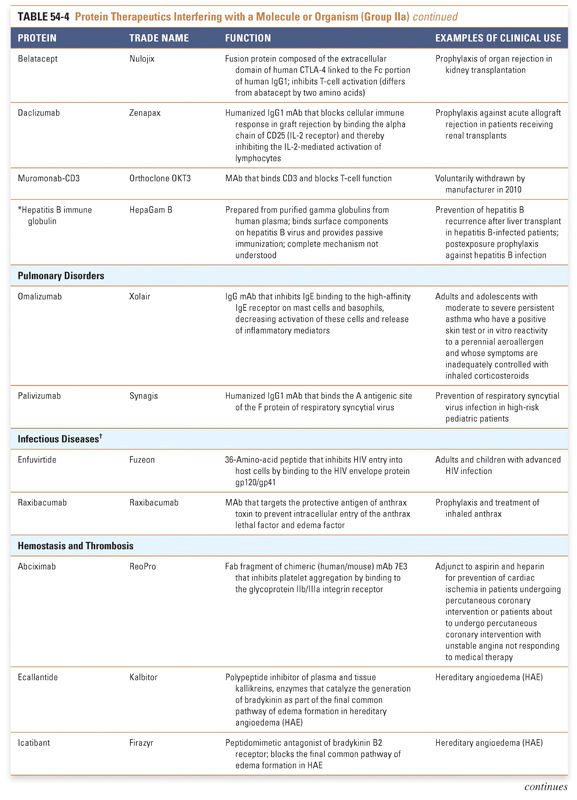
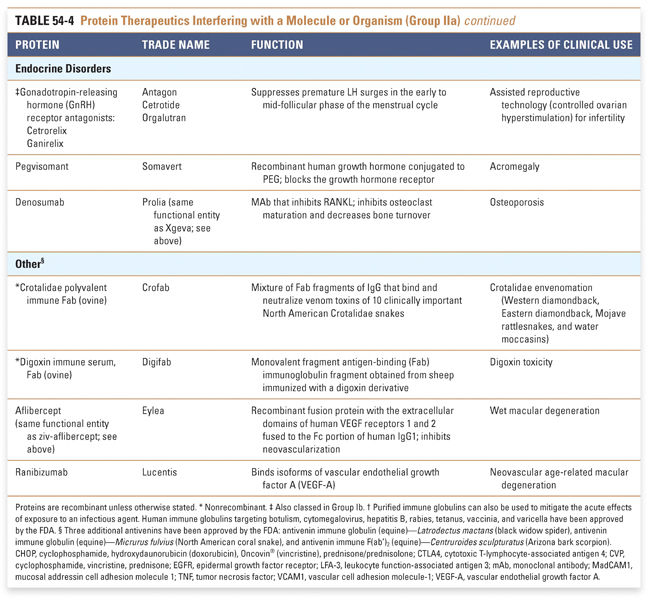
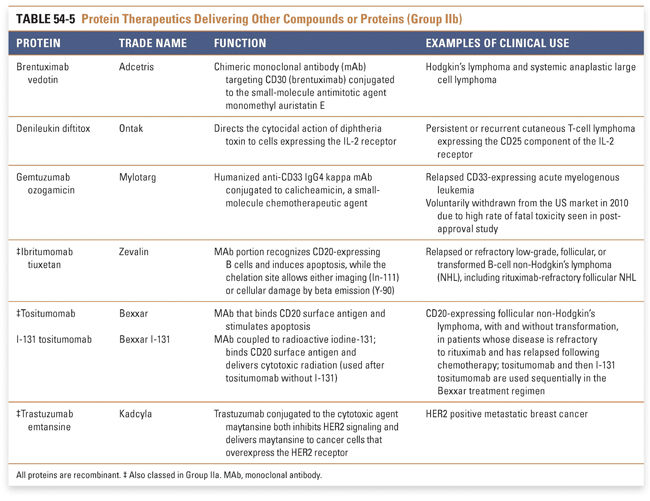
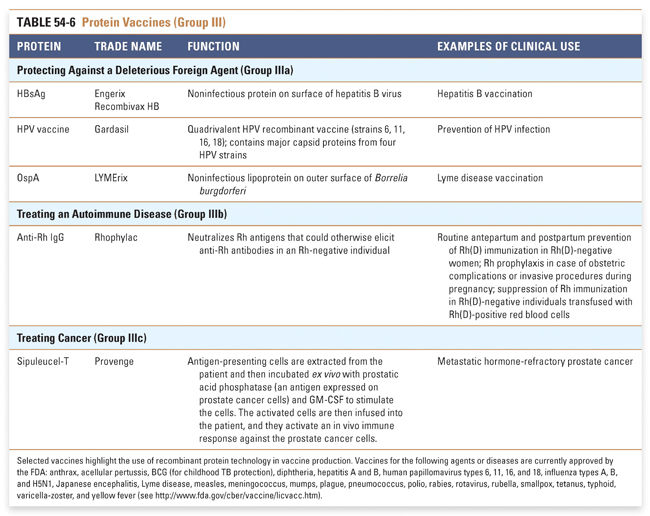
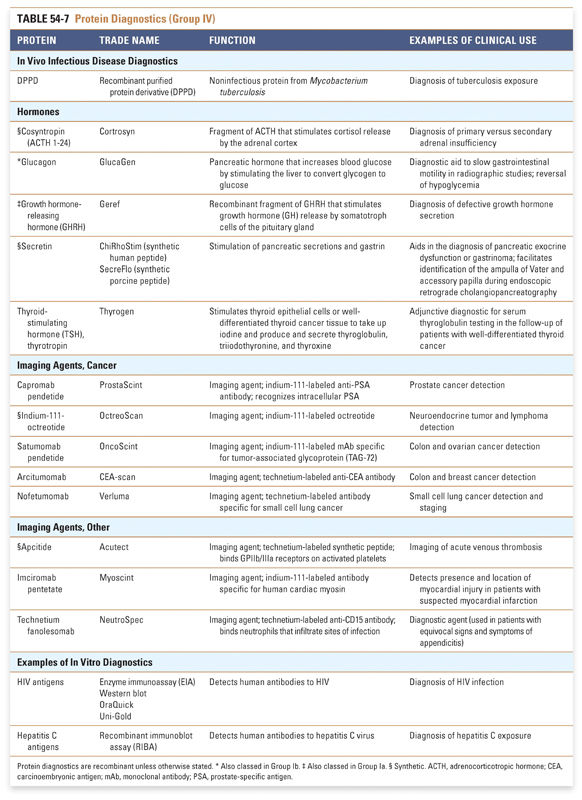
Group I: Enzymes and Regulatory Proteins
Protein therapeutics in this group function by a classic paradigm in which a specific endogenous protein is deficient, and the deficit is then remedied by treatment with exogenous protein. Protein therapeutics classified in Group Ia are used to replace a particular activity in cases of protein deficiency or abnormal protein production. These proteins are used in a range of conditions, from providing lactase in patients lacking this gastrointestinal enzyme to replacing vital blood-clotting factors such as factor VIII and factor IX in hemophiliacs. A classic example, as mentioned above, is the use of insulin for the treatment of diabetes. Another important example is in the treatment of cystic fibrosis, a common and often lethal genetic disorder. In this disease, defects in the chloride channel encoded by the CFTR
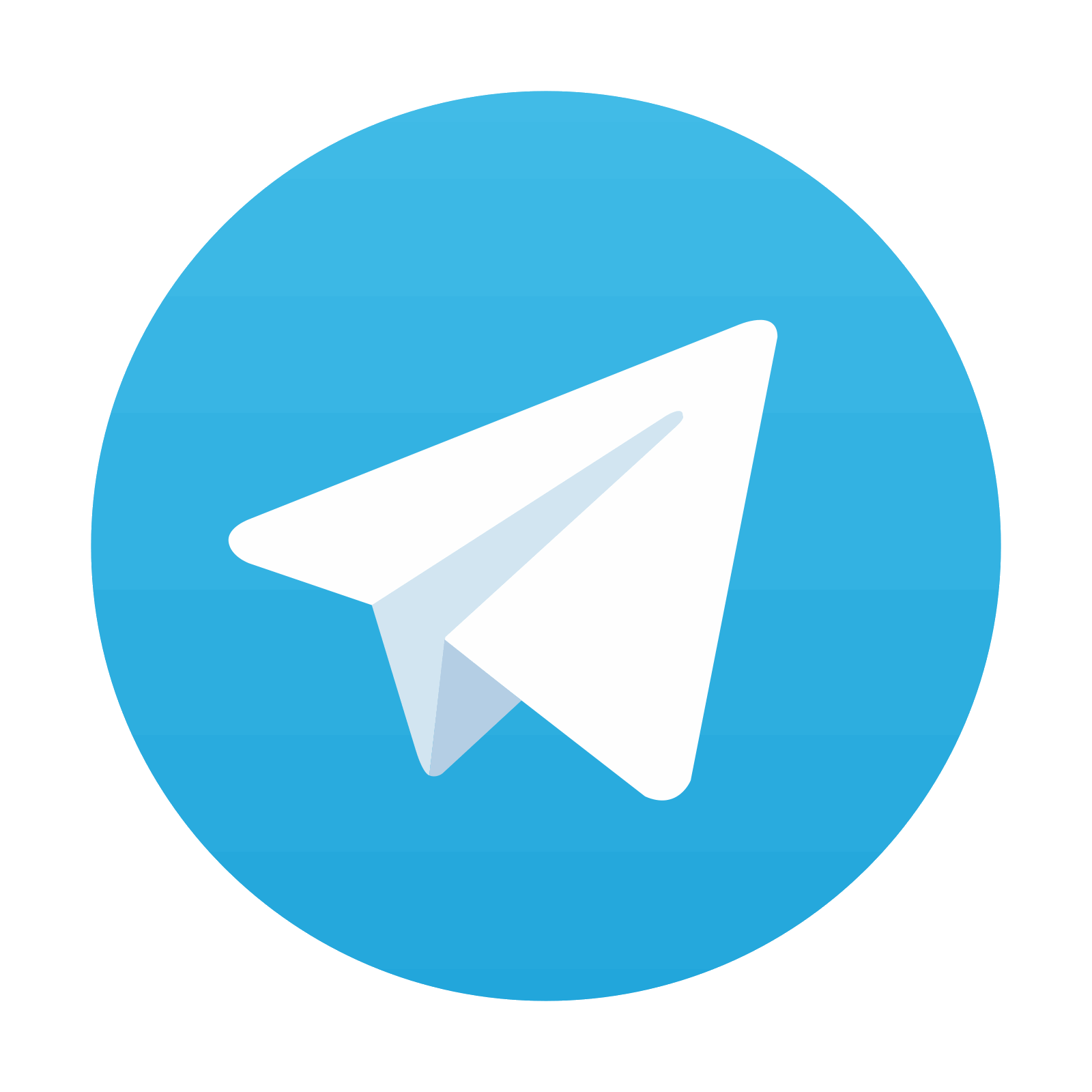
Stay updated, free articles. Join our Telegram channel
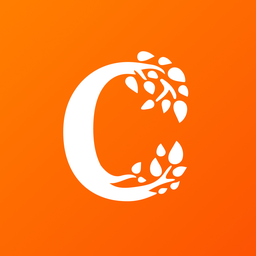
Full access? Get Clinical Tree
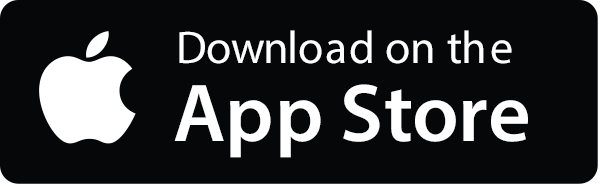
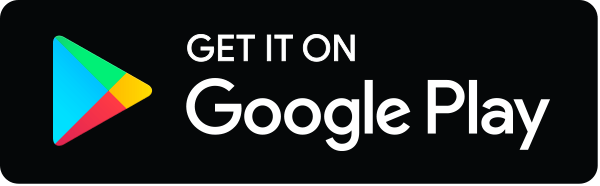