Chapter 49 During gestation, ≈90% of fetal body weight is water.22 Water is 80% of body weight for preterm infants and 70% for full-term infants. Water gradually decreases as percent of body weight, so that it accounts for 65% of body weight in older children, 60% in adolescents and adult males,22 and ≈55% for adult females. After middle age, the percentage of body water falls to ≈50%. As depicted in Figure 49-1, approximately two thirds of total body water (TBW) is distributed into the intracellular fluid (ICF) compartment, and one third exists in the extracellular fluid (ECF) compartment. The ICF and ECF compartments are physically separated by the cellular plasma membrane. The ECF may be subdivided into interstitial (≈75% of ECF) and intravascular (≈25% of ECF) fluid compartments, which are separated by the capillary endothelium. The average adult has ≈5 L blood volume (intravascular compartment) and a plasma volume of ≈3.0 L when the hematocrit is ≈40%. Although other clinically relevant ECF compartments (e.g., cerebrospinal fluid,31 urine) may be analyzed in the clinical laboratory, most laboratory tests used to determine hydration status and electrolyte and acid-base status are performed on samples from the intravascular compartment. The minimum daily requirement for water can be estimated from renal (1200 to 1500 mL in urine) and “insensible” losses (≈400 to 700 mL due to evaporation from the skin and respiratory tract). Activity, environmental conditions, and disease all have dramatic effects on daily water (and electrolyte) requirements. However, on average, an adult must take in ≈1.5 to 2.0 L of water daily to maintain fluid balance. Because primary regulatory mechanisms are designed to first maintain intracellular hydration status, imbalances in TBW are initially reflected in the ECF compartment. Table 49-1 lists common causes and clinical manifestations of expansion and contraction of the ECF compartment. TABLE 49-1 Causes and Clinical Manifestations of Changes in Extracellular Fluid (ECF) Volume The primary cationic (positively charged) electrolytes are sodium (Na+), potassium (K+), calcium (Ca2+), and magnesium (Mg2+), whereas the anions (negatively charged) include chloride (Cl−), bicarbonate ( TABLE 49-2 Electrolyte and Water Composition of Body Fluid Compartments* *All electrolyte values are expressed in mEq/L of fluid. Because the H2O content of plasma is ≈93% by volume, the corresponding electrolyte concentrations in plasma water are ≈10% higher. Note that the molar concentration of divalent ions is one half the depicted value. The extracellular compartment is composed of plasma and interstitial fluid. Plasma generally has a volume of 1300 to 1800 mL/m2 of body surface and constitutes approximately 5% of the body volume (≈3.5 L for a 66 kg subject). Generally, total body volume is derived from body mass by using an estimated body density of 1.06 kg/L. Table 49-2 describes the electrolyte composition of plasma. The mass concentration of water in normal plasma is about 0.933 kg/L, depending on the protein and lipid content (see “Electrolyte Exclusion Effect” in Chapter 28). Thus a concentration of sodium in the plasma of 140 mmol/L would correspond to a molality of sodium in plasma water of 150 mmol/kg H2O (140 mmol/L divided by 0.933 kg/L). The concentration of net protein ions in plasma is ≈12 mmol/L, with the charge mainly due to albumin, as the charge of globulins is negligible.8 Interstitial fluid is essentially an ultrafiltrate of blood plasma (see Figure 49-1). When all extracellular spaces except plasma are included, the volume accounts for about 26% (10.5 L) of the total body volume. Plasma is separated from the interstitial fluid by the endothelial lining of the capillaries, which acts as a semipermeable membrane and allows passage of water and diffusible solutes but not compounds of high molecular mass, such as proteins. The exchange of water between the interstitial and intravascular compartments is governed by Starling forces.22 The Starling formula demonstrates that the net movement of fluid across a capillary membrane is a function of membrane permeability and differences in hydrostatic and oncotic pressures on the two sides of the membrane. The “impermeability” to proteins is not absolute, and in some pathologic conditions causing “shock,” such as bacterial sepsis, the permeability of the vascular endothelium increases dramatically, resulting in leakage of albumin, a reduction in the effective circulating volume, and hypotension. If not aggressively treated with intravenous fluids and/or vasopressors, this condition can result in death as the result of decreased cerebral perfusion. The exact composition of ICF is extremely difficult to measure because of the relative unavailability of cells free of contamination. Although erythrocytes are easily accessible, it would be incorrect to make any generalizations based on the composition of these highly specialized cells. Data for ICF (see Table 49-2), therefore, are considered only approximations. The ICF constitutes ≈66% of the total body volume (see Figure 49-1). If solutions on two sides of a membrane contain different concentrations of ions that cannot freely move through the membrane (e.g., proteins), distribution of diffusible ions (e.g., electrolytes) at the steady state will be unequal, but the sum of the concentrations of ions in one compartment is equal to the sum of ions in the other compartment (Gibbs-Donnan law). Also, the law of electrical neutrality is obeyed for both compartments. An example of the uneven distribution of an ion in two compartments with different protein content (nondiffusible ions) is the concentration of chloride ions in plasma and cerebrospinal fluid (CSF). As a result of increased selectivity of the blood-brain barrier against proteins, Cl− ions are ≈15% higher in CSF to establish electrical and osmotic equilibrium.31 Calculations that demonstrate these principles can be found in the first edition of this textbook.27 Examination of Table 49-2 reveals that the electrolyte compositions of blood plasma and interstitial fluid (both ECFs) are similar, but their compositions differ markedly from that of ICF. The major ECF ions are Na+, Cl−, and In addition to the Na+/K+-ATPase, a ubiquitous Na+-H+ exchanger (often referred to as an antiporter) actively pumps H+ out of the ICF in exchange for Na+.20 This exchanger is critical for maintaining intracellular pH homeostasis in many cell types. At least six different isoforms of this transmembrane protein have been identified, and regulation and tissue distribution of these differ.20 Of particular importance is the role of this exchanger for acid-base regulation in renal tubular cells, as discussed later in this chapter. Disorders of Na+, K+, Cl−, and Disorders of Na+ homeostasis can occur because of excessive loss, gain, or retention of Na+, or as the result of excessive loss, gain, or retention of H2O. It is difficult to separate disorders of Na+ and H2O balance because of their close relationship in establishing normal osmolality in all body water compartments. As described in detail in Chapter 48, the primary organ for regulating body water and extracellular Na+ is the kidney. As a brief introduction to this section, it is important to remind the reader of the functions of healthy kidneys. In the proximal tubules, 70 to 80% of filtered Na+ is actively reabsorbed, with H2O and Cl− following passively to maintain electrical neutrality and osmotic equivalence. In the descending loop of Henle, H2O, but not electrolytes, is passively reabsorbed because of the high osmotic strength of interstitial fluid in the renal medulla. In the ascending loop of Henle, Cl− is reabsorbed actively, with Na+ following. At the level of the distal tubule, the first of the two primary Na+/H2O regulating processes occurs. Here, aldosterone stimulates the cortical collecting ducts to reabsorb Na+ (with water following passively) and secrete K+ (and to a lesser extent, H+) to maintain electrical neutrality. Aldosterone is produced by the adrenal cortex in response to angiotensin II derived via the action of renin. The secretion of renin by renal juxtaglomerular cells is stimulated by low chloride, by β-adrenergic activity, and by low arteriolar pressure.26 Thus when the kidneys are hypoperfused (as occurs when blood volume decreases, or when the renal arteries are obstructed), the distal tubules, under the influence of aldosterone, reclaim Na+. Further water regulation in the kidney occurs from the distal tubule through the collecting duct, where tubular permeability to H2O is under the influence of antidiuretic hormone (ADH) (see Chapters 48 and 53). ADH (also called vasopressin) is released by the posterior pituitary under the influence of baroreceptors in the aortic arch and of hypothalamic chemoreceptors that are responsive to circulating osmolality, which is primarily a reflection of Na+ concentration. When blood volume is decreased, or when plasma osmolality is increased, ADH is secreted, tubular permeability to H2O increases via aquaporins, and H2O is reabsorbed in an attempt to restore blood volume or to decrease osmolality. In contrast, when blood volume is increased or osmolality decreased, ADH secretion is inhibited, and more H2O is excreted in the urine (diuresis). Besides the kidney, the body’s only other mechanism for restoring Na+/H2O homeostasis is ingestion of H2O. Thirst is stimulated by decreased blood volume or by a hyperosmotic condition. It is important to remember that receptors that influence renal handling of Na+ and H2O, and thirst, sense changes only in the intravascular blood volume and not the total ECF. Furthermore, laboratory assessment of water and electrolyte disorders is made primarily from the blood volume (plasma). As discussed in subsequent sections, the clinician must assess the status of TBW and blood volume before interpreting laboratory values in the diagnosis of water and electrolyte disorders. The physical findings of these disorders are every bit as important as the laboratory values (see Table 49-1). Hyponatremia is defined as a decreased plasma Na+ concentration (<130 to 135 mmol/L). Hyponatremia typically manifests clinically as nausea, generalized weakness, and mental confusion at values <120 mmol/L, ocular palsy at <110 mmol/L, and severe mental impairment at between 90 and 105 mmol/L.25 The rapidity of development of hyponatremia influences the Na+ concentrations at which symptoms develop [i.e., clinically apparent symptoms may manifest at higher Na+ concentrations (≈125 mmol/L) when hyponatremia develops rapidly].25 It is important to note that symptoms are due to changes in osmolality rather than to the Na+ concentration per se. Central nervous system (CNS) symptoms are due primarily to movement of H2O into cells to maintain osmotic balance and subsequent swelling of CNS cells. Hyponatremia can be hypo-osmotic, hyperosmotic, or isosmotic. Thus, measurement of plasma osmolality is an important initial step in the assessment of hyponatremia. Of these, the most common form is hypo-osmotic hyponatremia. Figure 49-2 describes an algorithm for laboratory measurements and physical examination findings in the differential diagnosis of plasma Na+ <135 mmol/L. Depletional hyponatremia (excess loss of Na+) is almost always accompanied by loss of ECF water, but to a lesser extent than Na+ loss. This occurs because thirst leads to ingestion of water, which obviously is more hypotonic than the lost fluids. Hypovolemia is apparent in the physical examination (orthostatic hypotension, tachycardia, decreased skin turgor). If urine Na+ is low (generally <10 mmol/L), the loss is extrarenal (see Figure 49-2), as the kidneys are properly retaining filtered Na+ in response to aldosterone, which is stimulated by hypovolemia. Causes of extrarenal loss of Na+ in excess of H2O include losses from the gastrointestinal tract or skin (see Figure 49-2). In hypo-osmotic hyponatremia with a normal volume status, the most common causes are the syndrome of inappropriate ADH (SIADH), primary polydipsia, and hypothyroidism (see Figure 49-2). SIADH is usually a result of ectopic or otherwise “inappropriate” ADH production arising from a variety of conditions6 (see Chapters 48 and 53) and results in excessive H2O retention. SIADH is often diagnosed when urine osmolality is greater than plasma osmolality, but only when renal, adrenal, and thyroid function are normal. In cortisol insufficiency, hyponatremia can occur as a result of increased cortisol-releasing hormone, which stimulates vasopressin release.29 Hypothyroidism impairs free H2O excretion. Finally, euvolemic hyponatremia can be found in polydipsia when water intake is greater than the renal capacity to excrete excess H2O. This is most often the result of psychiatric illness, but diseases that cause hypothalamic disorders, such as sarcoidosis, may also cause polydipsia by altering the thirst reflex (see Figure 49-2). Hyponatremia that occurs in the presence of increased quantities of other solutes in the ECF is the result of an extracellular shift of water or an intracellular shift of Na+ to maintain osmotic balance between ECF and ICF compartments. The most common cause of this type of hyponatremia is severe hyperglycemia (see Figure 49-2). As a general rule, Na+ is decreased by ≈1.6 to 2.4 mmol/L for every 100 mg/dL increase in glucose above 100 mg/dL.30 Correction of hyperglycemia will restore normal blood Na+. If the measured Na+ concentration in plasma is decreased, but measured plasma osmolality, glucose, and urea are normal, the most likely explanation is pseudohyponatremia caused by the electrolyte exclusion effect (see Chapter 28). This occurs when Na+ is measured by an indirect ion-selective electrode (and flame photometry in the past) in patients with severe hyperlipidemia. It can also occur when osmotically active agents such as mannitol and glycine are used in irrigation fluids for transurethral resection of the prostate (TURP) and endometrial ablation. Hyponatremia must be corrected cautiously because too rapid correction can lead to brain demyelination. The pons is particularly sensitive to this, and rapid correction can lead to central pontine myelinolysis. Current recommendations are to increase Na+ by 0.5 to 2.0 mmol/L/h and not to exceed a total increase in Na+ greater than 18 to 25 mmol over 48 hours.30 The rate of recommended Na+ increase is dependent on symptoms and length of time the patient is hyponatremic. Hypernatremia (plasma Na+ >150 mmol/L) is always hyperosmolar. Symptoms of hypernatremia are primarily neurologic (because of neuronal cell loss of H2O to the ECF) and include tremors, irritability, ataxia, confusion, and coma.25 As with hyponatremia, the rapidity of development of hypernatremia will determine the plasma Na+ concentration at which symptoms occur. Acute development may cause symptoms at 160 mmol/L, although in chronic hypernatremia, symptoms may not occur until Na+ exceeds 175 mmol/L. In chronic hypernatremia, the intracellular osmolality of CNS cells will increase to protect against intracellular dehydration. Because of this, rapid correction of hypernatremia can cause dangerous cerebral edema, as CNS cells will take up too much water if the ICF is hyperosmotic when normonatremia is achieved.25 Hypernatremia arises in the setting of (1) hypovolemia (excessive water loss or failure to replace normal water losses), (2) hypervolemia (a net Na+ gain in excess of water gain), or (3) normovolemia. Again, assessment of TBW status by physical examination and measurement of urine Na+ and osmolality are important steps in establishing a diagnosis (Figure 49-3). Hypernatremia in the setting of decreased ECF is caused by renal or extrarenal loss of hypo-osmotic fluid, leading to dehydration. Thus once hypovolemia is established by physical examination, measurement of urine Na+ and osmolality is used to determine the source of fluid loss. Patients who have large extrarenal losses will have concentrated urine (often >800 mOsmol/L) with low urine Na+ (<20 mmol/L), reflecting a proper renal response to conserve Na+ and water to restore ECF volume. Extrarenal causes include diarrhea, skin losses (burns, fever, or excessive sweating), and respiratory losses coupled with failure to replace the water. When gastrointestinal loss is excluded, and the patient has normal mental status and access to H2O, a hypothalamic disorder (tumor or granuloma) should be suspected, because the normal thirst response should always replace insensible water losses.25 Hypernatremia in the presence of normal ECF volume is often a prelude to hypovolemic hypernatremia. Insensible losses through the lung or skin again must be suspected and are characterized by concentrated urine as the kidneys conserve water. Another cause of normovolemic hypernatremia is water diuresis, which is manifested by polyuria (see Figure 49-3). The differential for polyuria (generally defined as >3 L urine output/d) is a water or solute diuresis. Solute diuresis is exemplified by the osmotic diuresis of diabetes mellitus and generally is characterized by urine osmolality >300 mOsmol/L and hyponatremia (see previous discussion in this chapter). Water diuresis, a manifestation of diabetes insipidus (DI), is characterized by dilute urine (osmolality <250 mOsmol/L) and slight hypernatremia.25 DI can be central or nephrogenic.21 Central DI is due to decreased or absent ADH secretion resulting from head trauma, hypophysectomy, pituitary tumor, or granulomatous disease. Nephrogenic DI is due to renal resistance to ADH as a result of drugs (e.g., lithium, demeclocycline, amphotericin, propoxyphene); sickle cell anemia and Sjögren syndrome, which affect collecting duct responsiveness to ADH; or, more rarely, mutant ADH receptors.2 Central DI usually is treated with vasopressin, whereas nephrogenic DI is treated by discontinuing the drug or providing easy access to water, which will maintain normonatremia. The total body potassium of a 70 kg subject is ≈3.5 mol (40 to 59 mmol/kg), of which only 1.5 to 2% is present in the ECF. Nevertheless, plasma K+ is often a good indicator of total K+ stores, unless abnormal K+ is due to abnormal cellular shifts. Disturbance of K+ homeostasis has serious consequences. For example, a decrease in extracellular K+ (hypokalemia) is characterized by muscle weakness, irritability, and paralysis. Plasma K+ concentrations less than 3.0 mmol/L are often associated with marked neuromuscular symptoms and indicate a critical degree of intracellular depletion. At lower concentrations, tachycardia and cardiac conduction defects are apparent by electrocardiogram (flattened T waves) and can lead to cardiac arrest.25 High extracellular K+ (hyperkalemia) concentrations may produce symptoms of mental confusion, weakness, tingling, flaccid paralysis of the extremities, and weakness of the respiratory muscles.25 Cardiac effects of hyperkalemia include bradycardia and conduction defects evident on the electrocardiogram as prolonged PR and QRS intervals and “peaked” T waves. Prolonged severe hyperkalemia >7.0 mmol/L can lead to peripheral vascular collapse and cardiac arrest. Symptoms are almost always present at K+ concentrations >6.5 mmol/L. Concentrations >10.0 mmol/L in most cases are fatal, but as with Na+, symptoms vary with the rapidity of onset. Causes of hypokalemia (plasma K+ <3.5 mmol/L) are classified as redistribution of extracellular K+ into ICF, or true K+ deficits, caused by decreased intake or loss of potassium-rich body fluids (Figure 49-4). Intracellular redistribution of K+ is illustrated by the fall in plasma K+ that occurs following insulin therapy for diabetic hyperglycemia when cells take up K+ as a consequence of glucose transport. Redistribution hypokalemia is also a feature of alkalosis, in which K+ moves from ECF into cells as increased H+ alters activity of the Na+/K+-ATPase. In addition, renal conservation of H+ in the distal tubule occurs at the expense of K+. Hypokalemia is highly prevalent in cancer patients. Pseudohypokalemia is a feature of acute leukemia. The elevated white blood cell count can cause time-dependent transport of K+ into leukemic cells after the blood sample is drawn. Additionally, use of myelopoietic growth factors following chemotherapy can lead to rapid K+ uptake by new cells.17 Other causes of hypokalemia in cancer patients include tumors (small cell lung, pheochromocytoma, islet cell, medullary thyroid) that produce adrenocorticotropic hormone (ACTH), resulting in increased cortisol and renal loss of K+. Other causes of intracellular redistribution are listed in Figure 49-4. Hypokalemia reflecting true total body deficits of K+ can be classified into renal and nonrenal losses, based on daily excretion of K+ in the urine (see Figure 49-4). If urine excretion of K+ is <30 mmol/d, it can be concluded that the kidneys are properly functioning and are attempting to reabsorb K+ as appropriate in a hypokalemic setting. The cause may be decreased K+ intake or extrarenal loss of K+-rich fluid. Situations of decreased intake include chronic starvation and postoperative intravenous fluid therapy with K+-poor solutions. Gastrointestinal loss of K+ occurs most commonly with diarrhea and loss of gastric fluid through vomiting or prolonged nasogastric suction. Urine excretion exceeding 25 to 30 mmol/d in a hypokalemic setting is inappropriate and indicates that the kidneys are the primary source of lost K+. Renal losses of K+ may occur during the diuretic (recovery) phase of acute tubular necrosis and during states of excess mineralocorticoid (primary or secondary aldosteronism) or glucocorticoid (Cushing syndrome) when the distal tubules increase Na+ reabsorption and K+ excretion. Renal loss of K+ is also caused by thiazide and loop diuretics.16 In addition to redistribution of K+ into cells in an alkalotic setting, K+ can be lost from the kidneys in exchange for reclaimed H+ ions. This cause of true hypokalemia will be evident in low urine Cl− and an alkaline urine. In cancer patients, increased renal K+ loss is due to chemotherapy (e.g., cisplatin)-induced nephron and tubular damage.17 Hyperkalemia (plasma K+ >5.0 mmol/L) is a result of (singly or in combination) (1) redistribution, (2) increased intake, or (3) increased retention. In addition, preanalytical conditions—such as hemolysis, thrombocytosis (>106/µL), and leukocytosis (>105/µL)—have been known to cause marked pseudohyperkalemia, as described in detail in Chapter 28 (Figure 49-5). The transfer of intracellular K+ into ECF invariably occurs in acidosis as K+ shifts outward as the result of pH-induced changes in Na+/K+-ATPase activity. As a general rule, K+ concentrations can be expected to rise 0.2 to 0.7 mmol/L for every 0.1 unit drop in pH. When acidosis is corrected, normokalemia will be restored rapidly. Extracellular redistribution of K+ may also occur in (1) tissue hypoxia; (2) insulin deficiency (e.g., diabetic ketoacidosis); (3) massive intravascular hemolysis; (4) severe burns; (5) violent muscular activity, as in status epilepticus; (6) rhabdomyolysis; and (7) tumor lysis syndrome. Finally, important iatrogenic causes of redistribution hyperkalemia include digoxin toxicity and β-adrenergic blockade, especially in patients with diabetes or on dialysis.25 When glomerular filtration or renal tubular function is decreased, hyperkalemia may be precipitated by intravenous infusion of K+. When renal function is normal, overtreatment with K+ solutions is unlikely to produce hyperkalemia, because renal capacity is more than adequate to excrete the excess K+. Indeed, in the absence of severe renal failure, hyperkalemia is seldom prolonged. Decreased excretion of K+ in moderate and acute renal disease and end-stage renal failure (with oliguria or anuria) are the most common causes of prolonged hyperkalemia (see Figure 49-5). Hyperkalemia occurs along with Na+ depletion in adrenocortical insufficiency (e.g., Addison disease) because diminished Na+ reabsorption results in decreased tubular K+ secretion. Drugs that block the production of aldosterone, such as inhibitors of angiotensin-converting enzyme (ACE inhibitors; e.g., lisinopril), nonsteroidal anti-inflammatory drugs, and angiotensin II receptor blockers, may also cause hyperkalemia. Excess administration of potassium-sparing diuretics that block distal tubular K+ secretion (e.g., triamterene, spironolactone) commonly causes hyperkalemia.11 In cancer patients, hyperkalemia can be caused by adrenal insufficiency due to metastases to the adrenal glands, nephrotoxic chemotherapy agents (e.g., mitomycin-C, methotrexate, platinum compounds), postrenal obstruction, or tumor lysis syndrome.17 Treatment of hyperkalemia includes agents that increase cellular uptake of K+ such as calcium gluconate, glucose and insulin, sodium bicarbonate, and β2-adrenergic agonists. Potassium can be removed from the circulation with the use of K+-losing diuretics, cation-exchange resins, and finally hemodialysis.17 Increased plasma Cl− concentration, similar to increased Na+ concentration, occurs with dehydration, prolonged diarrhea with loss of sodium bicarbonate, DI, and overtreatment with normal saline solutions, which have a Cl− content of 154 mmol/L. In fact, mounting evidence suggests that use of saline (NaCl) solution for maintenance, intraoperative, and resuscitative therapy can result in a host of hyperchloremia-induced side effects.10 Thus, there is a movement among the surgical community toward more physiologic solutions such as Ringer’s lactate and Hartmann’s solution.10 A rise in Cl− concentration may also be seen in respiratory alkalosis because of renal compensation for excreting The pH of a solution is defined as the negative logarithm of the hydrogen ion activity (pH = −log aH+). Thus pH is a dimensionless quantity, such that a decrease in one pH unit represents a tenfold increase in H+ activity. Potentiometric determinations of blood pH measure H+ activity and not H+ concentration, although the activity is assumed to equal the concentration. The average pH of blood (7.40) corresponds to a hydrogen ion concentration of 40 nmol/L, but this assumes an activity coefficient of 1. The relationship between hydrogen ion activity and pH is illustrated in Figure 49-6. This relationship is inverse and is obviously nonlinear. Expressing the acidity of blood in terms of its hydrogen ion concentration in nanomoles per liter (nmol/L) has the advantage of avoiding logarithmic transformations when acid-base calculations are performed. Even though it would be consistent with how other ion concentrations are expressed, this method has not gained widespread use in the United States.
Physiology and Disorders of Water, Electrolyte, and Acid-Base Metabolism
Total Body Water—Volume and Distribution
Clinical Manifestations
Causes
ECF loss
Thirst, anorexia, nausea, lightheadedness, orthostatic hypotension, syncope, tachycardia, oliguria, decreased skin turgor and “sunken eyes,” shock, coma, death
Trauma (and other causes of acute blood loss), “third-spacing” of fluid (e.g., burns, pancreatitis, peritonitis), vomiting, diarrhea, diuretics, renal or adrenal (i.e., sodium wasting) disease
ECF gain
Weight gain, edema, dyspnea (due to pulmonary edema), tachycardia, jugular venous distention, portal hypertension (ascites), esophageal varices
Heart failure, cirrhosis, nephrotic syndrome, iatrogenic (intravenous fluid overload)
Water and Electrolytes—Composition of Body Fluids
), phosphate (
,
), sulfate (
), organic ions such as lactate, and negatively charged proteins. Electrolyte concentrations of the body fluid compartments are shown in Table 49-2. Na+, K+, Cl−, and
in the plasma or serum are commonly analyzed in an electrolyte profile, because their concentrations provide the most relevant information about the osmotic, hydration, and acid-base status of the body. Although hydrogen ion (H+) is a cation, its concentration is approximately 1 million–fold lower in plasma than the major electrolytes listed in Table 49-2 (10−9 mol/L vs. 10−3 mol/L) and thus is negligible in terms of osmotic activity.
Extracellular and Intracellular Compartments
Plasma
Interstitial Fluid
Intracellular Fluid
Reasons for Composition Differences of Body Fluids
Gibbs-Donnan Equilibrium
Distribution of Ions by Active and Passive Transport
, but in ICF, the main ions are K+, Mg2+, organic phosphates, and protein. This unequal distribution of ions is due to active transport of Na+ from inside to outside the cell against an electrochemical gradient. This process requires energy supplied by metabolic processes in the cell (e.g., glycolysis). An active sodium pump deriving its energy from adenosine triphosphate (ATP) is present in most cell membranes and frequently is coupled with transport of K+ into the cell. The Na+/K+ pump is a heterodimer consisting of a catalytic transmembrane α-subunit that is about 1000 amino acid residues long, and an associated, smaller β-subunit.13 The internal surface has a catalytic binding site for ATP and a binding site for Na+. The external surface has a binding site for K+.
Electrolytes
will now be separately considered, even though disorders of electrolyte and water homeostasis need a systematic evaluation.18
Sodium
Hyponatremia
Hypo-Osmotic Hyponatremia
Hyperosmotic Hyponatremia
Isosmotic Hyponatremia
Hypernatremia1
Hypovolemic Hypernatremia
Normovolemic Hypernatremia
Potassium
Hypokalemia
Redistribution
True Potassium Deficit
Hyperkalemia
Redistribution
Potassium Retention
Chloride
Hyperchloremia
.
Acid-Base Physiology
Acid-Base Balance and Acid-Base Status
Acid-Base Parameters—Definitions and Abbreviations
pH and pK
Stay updated, free articles. Join our Telegram channel
Full access? Get Clinical Tree
Physiology and Disorders of Water, Electrolyte, and Acid-Base Metabolism
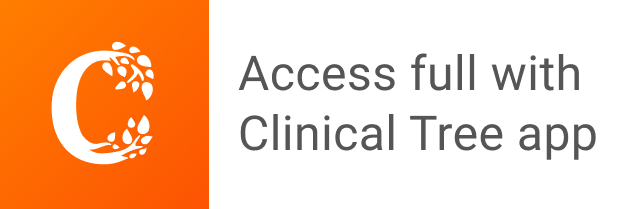