Pharmacogenomics and Pharmacogenetics for Infectious Diseases
LEARNING OBJECTIVES
INTRODUCTION
The concept of “chemical individuality” was developed in 1908 by Sir Archibald Garrod in 1908. He described the incidence of toxicity in a small proportion of individuals with a dose of a drug that had no toxic effects in the majority of the patients. The first example of a pharmacogenetic study probably is an observation that “taste blindness” was inherited in an autosomal recessive manner and that the characteristic varied in different ethnic groups.1
The recognition of pharmacogenomics as a distinct discipline occurred in the 1950s in the context of antimalarial dugs. Treatment with antimalarial drugs such as primaquine resulted in development of a hemolytic crisis in a high proportion of African American soldiers. Variants in glucose 6-phosphate dehydrogenase (G6PD) were identified to be responsible for this toxicity. The term “pharmacogenetics” was coined by Friedrich Vogel in 1959.
INFLUENCE OF VIRAL GENETICS ON DRUG RESPONSE
Drug response can be influenced by the viral genome as well as the host genome. Thus, both viral and host genome considerations are important in optimizing therapy in viral diseases.
Viral Genotype as Predictor of Response
Viral genotype can be a predictor of response. An example is that of treatment of chronic hepatitis C infection with peginterferon (PEG-IFN) and ribavirin (RBV). Response rates to PEG-IFN and RBV vary depending on HCV genotype: about 40–50% of the patients infected with genotype 1 virus treated for 48 weeks with PEG-IFN and RBV reach sustained virologic response (SVR), whereas 70–80% of patients with genotype 2 or 3 virus attain SVR after only 24 weeks of PEG-IFN and RBV therapy.2
Viral Genetics and Resistance to Antiviral Treatments
Viral genome also is an important consideration in antiretroviral therapy. Certain sequences in the viral genome confer resistance to antiretroviral treatments. In addition, resistance to an antiretroviral drug can develop during treatment causing treatment failure in patients infected with agents such as HIV. Contributors to the development of antiretroviral drug resistance are errors in copying viral RNA into DNA that include base substitutions, coupled with high rate of replication of HIV viruses. In addition, multiple variants of the virus might be present in HIV-infected patients and these could have differing sensitivities to antiretroviral therapy that make successful treatment of HIV infection challenging. Thus, an understanding of the mechanisms leading to resistance and development of strategies to identify and avoid/overcome drug resistance can lead to successful therapy. HIV drug resistance testing is one of the powerful tools that is currently being used to help clinicians tailor combination therapy to HIV patients.3,4
Viral Genetics, HIV Tropism, and Selection of Patients for Antiviral Therapy
The tropism of HIV viruses is important in determining whether a targeted therapeutic, such as maraviroc, should be prescribed to HIV patients. The European Consensus Group on clinical management recently recommended V3 (HIV third hypervariable loop) population genotyping as one of the methods for tropism testing based on recent data.5
INFLUENCE OF HOST GENETICS ON DRUG RESPONSE
Drugs are absorbed, distributed, metabolized, and eliminated in the host and mechanisms involved in these steps are likely to influence the outcome. This is particularly true for drugs with narrow therapeutic indices and for drugs that are metabolized and/or transported by polymorphic proteins that can differentially influence the rate at which the drug is metabolized or transported. Several antiretroviral drugs fit this description.6
Pharmacogenomics and Disease Progression
Variations in the host genome could influence disease progression through impacting viral–host interaction, which is the first step in viral infections. An example is that of a mutation (Δ32, deletion of 32 base pair) in the CCR5 receptor that results in formation of a truncated protein that is not transported to the cell surface. A strong, but incomplete, resistance to HIV infection has been reported in individuals who are homozygous for the Δ32 allele and delayed progression to disease has been reported in individuals heterozygous for the allele.7 In addition, several genetic polymorphisms in the CCR5 promoter region have been reported to affect HIV transmission or disease progression.8
PHARMACOGENOMICS CAN ALTER PHARMACOKINETICS AND IMPACT DRUG DISPOSITION AND THERAPY OF DRUGS
Interindividual differences in patient response to treatment make it difficult to predict the safety and efficacy with standard doses of drugs. Most of the drugs appear to have the expected therapeutic effect in only 50% of the patients treated with the standard regimen. The patients who do not have the expected response are either underdosed with less/no therapeutic benefit or overdosed with associated drug toxicity. This poses a serious concern, particularly with drugs that have narrow therapeutic indexes.9
Genetic variations in the drug-metabolizing enzymes or transporter proteins are reflected in the enzyme activities or in the transporter activities, respectively.10 Four major phenotypes of drug-metabolizing enzymes can be identified by phenotyping or genotyping as extensive metabolizer (EM), poor metabolizer (PM), intermediate metabolizer (IM), and ultrarapid metabolizer (UM). Rapid clearance rates caused by patients with UM genotype could result in suboptimal concentrations of drugs leading to lower efficacious response to drugs. On the other hand, slower clearance due to PM or IM genotype may lead to drug accumulation and toxicity. With a prodrug, the effect of the metabolizer genotype will be reversed, where the PM genotype is associated with lower efficacy and the UM genotype is associated with toxicity. Knowledge of the identity of the metabolizing enzyme(s) and transporter(s) associated with the drug in combination with the knowledge of genetic variations in these proteins in an individual can help identify the appropriate dose required by the individual.
Examples showing impact of genetic variations in drug-metabolizing enzymes and transporters are described below.
PHASE 1 ENZYMES (CYTOCHROME P450)
Cytochrome P450 2B6 (CYP2B6)
CYP2B6 was initially not thought to be important in drug metabolism, but recent studies revealed the relevance of this enzyme in the metabolism of the anticancer drugs cyclophosphamide and ifosfamide, and the anti-HIV drugs efavirenz (EFV) and nevirapine (NVP).
The human CYP2B6 gene is highly polymorphic. Variant alleles are associated with lower expression such as CYP2B6*6, CYP2B6*16, and CYP2B6*18. Of these, CYP2B6*6 is common in several different populations including Chinese (20–30% frequency), whereas CYP2B6*16 and CYP2B6*18 are common in black subjects where the allele frequency is relatively high (7–9%).
In clinical studies, subjects homozygous for the alleles CYP2B6*6, CYP2B6*16, and CYP2B6*18 exhibit lower capacity for metabolism of CYP2B6 substrates such as EFV.11 In addition, the CYP2B6*6 allele appears to cause both high and low activity of CYP2B6 in different studies. It is possible that the 516G>T and 785A>G mutations, causing the amino acid substitutions Q172H and K262R, are linked to other mutations, giving rise to specific haplotypes that are associated with high or low activity of CYP2B6. Also, the CYP2B6*4 allele can cause higher Vmax values in vivo.12
Overall, there is a marked interindividual variability in the CYP2B6 activity. These interindividual differences in CYP2B6 expression may lead to variable systemic exposure, and perhaps response, to drugs metabolized by CYP2B6.
CYP2B6 and Efavirenz
The antiretroviral drug EFV is a non-nucleoside reverse transcriptase inhibitor (NNRTI) and an integral part of the combination therapy for HIV patients. EFV has a relatively narrow therapeutic index and its plasma concentration displays large interindividual variability. CYP2B6 is primarily responsible for EFV metabolism, to the major metabolite 8-hydroxy-EFV.13 Therefore, pharmacogenetics has great potential to influence the clinical use of this drug.
Elevated plasma concentrations of EFV cause CNS-related side effects such as dizziness and depression. This can contribute to discontinuation, poor adherence, and eventual therapeutic failure. Among the identified polymorphic alleles, CYP2B6*6, predominantly the 516G>T mutation, is associated with elevated EFV plasma concentrations and CNS-related side effects in several studies.14
The high frequency of the 2B6*6 allelic expression in a variety of ethnic populations suggests that CYP2B6 genotyping be used as a tool for EFV dose choice and adjustment. Genotype-specific dosing of EFV, in a Japanese cohort study, led to a decrease in EFV dose by 33% or 66% and a decrease in plasma concentrations proportionate to the reduction in dose. Patients maintained therapeutic effectiveness and their CNS-related symptoms improved.15
Although the role of CYP2B6 polymorphisms in EFV metabolism is now firmly established, data regarding drug–drug interactions between EFV and concomitant therapeutics are limited. Of particular concern is the interaction between EFV and rifampicin, where it appears that induction of EFV metabolism by rifampicin may be abrogated or even reversed by CYP2B6 polymorphisms, therefore leading to a paradoxical increase in EFV in patients with these polymorphisms.16 Increasing the dose of EFV as recommended in some treatment guidelines may therefore be disastrous in these patients.
CYP2B6 and Nevirapine
NVP is another NNRTI used to treat HIV infection in combination with other anti-HIV drugs. In human liver microsomes, NVP is oxidized to 2, 3-, 8-, and 12-hydroxynevirapine. In cDNA-expressed human CYPs, 2- and 3-hydroxynevirapine were exclusively formed by CYP3A4/3A5 and 2B6, respectively. Multiple cDNA-expressed CYPs produced 8- and 12-hydroxynevirapine, although CYP2D6 and 3A4 primarily catalyzed their formation, respectively. For the formation of 12-OH-nevirapine, CYP3A5, 2D6, and 2C9 (minor) also play a role, while CYP2A6, 2B6, and 3A4 catalyzed 8-OH-nevirapine formation with a low activity. Overall, NVP is principally metabolized by CYP3A4 and 2B6,17 and this complicated metabolism is reflected in the equivocal results for the role of CYP2B6 in NVP metabolism.
CYP2B6 516T/T genotype was associated with greater (1.7-and 1.8-fold) plasma levels of NVP in HIV-infected patients.18 No association was observed between the other polymorphisms in MDR1 3435C>T and 2677G>T, CYP3A4*1B and CYP3A5*3, and NVP concentrations in these patients.19
The 983T>C SNP, part of the CYP2B6*18 allele, was also associated with NVP plasma concentration. However, 1459C>T was not associated with plasma concentrations of NVP.20 However, other studies showed that CYP2B6 516/983 metabolizer status was not associated with NVP levels.21,22
Pharmacogenetics may also affect anti-HIV treatment outcomes. Children with the 516T/T genotype had decreased clearance and significant increase in CD4+ T-cell percentage, compared with those with the G/G and G/T genotypes, from baseline to week 12.23
Pharmacogenetics may also affect NVP toxicities. Haas et al. studied the association between MDR1, CYP2B6, and cytochrome P450 3A (CYP3A) polymorphisms and NVP hepatotoxicity in a randomized study in South Africa. Among the polymorphisms studied, only MDR1 3435C>T was significantly associated with decreased risk of hepatotoxicity (risk ratio = 0.30).24 This polymorphism was also found to correlate with hepatotoxicity in Mozambique. Four other SNPs in CYP2B6 and CYP3A5 correlated with transaminase levels.25 In a case–control study, cutaneous reactions were associated with CYP2B6 516G>T (OR 1.66), HLA-Cw*04 (OR 2.51), and HLA-B*35 (OR 3.47). Hepatic AEs were associated with HLA-DRB*01 (OR 3.02), but not CYP2B6 genotypes. Associations differed by population, at least in part reflecting allele frequencies.26 While promising, the effect size is much smaller than other recognized associations such as HLA-B*5701 and abacavir.
Cytochrome P450 2C19 (CYP2C19)
CYP2C19 is responsible for the metabolism of approximately 10% of commonly used drugs. These include proton pump inhibitors, tricyclic antidepressants, selective serotonin reuptake inhibitors, moclobemide, benzodiazepines, barbiturates, phenytoin, bortezomib, voriconazole, selegiline, nelfinavir, and proguanil.
CYP2C19 is a highly polymorphic enzyme with wide variation of distribution in various ethnic groups. The Asian population has a much greater frequency of CYP2C19 PMs (12–23%), when compared with Caucasians (1–6%) and black Africans (1–7.5%). The frequency of PMs in African, African American, and Middle Eastern populations is very similar to Caucasians. Indigenous populations, such as the Canadian Indians and the Australian Aboriginals, have a high frequency of PMs, which is similar to that of the Asian population. Further details about CYP2C19 are available in other chapters of this textbook.
CYP2C19 and Voriconazole
Voriconazole is a second-generation broad-spectrum triazole antifungal agent, exhibiting potent activity against a broad range of significant pathogens, including Aspergillus, Candida, Scedosporium, and Fusarium species. Voriconazole is extensively metabolized by the liver, with less than 2% of the dose excreted unchanged. N-oxidation of the fluoropyrimidine ring, its hydroxylation, and hydroxylation of the adjacent methyl group are known pathways of voriconazole oxidative metabolism, with the N-oxide being the major circulating metabolite in humans. Voriconazole is mainly metabolized by CYP2C19 and 2C9 and, to a lesser extent, by CYP3A4 and FMO1.
The considerable interindividual variability in the pharmacokinetics of voriconazole is partially due to CYP2C19 polymorphism, with PMs having 2- to 6-fold higher AUCs than EMs.27 In a study with 28 pediatric patients, heterozygous EMs and PMs had a 46% lower clearance of voriconazole than homozygous EMs.28 Therefore, PMs may be predisposed to concentration-dependent side effects such as hepatotoxicity. However, there was no significant relationship between CYP2C19 polymorphisms and serum liver enzyme levels in patients treated with voriconazole.29 On the other hand, UMs had significantly lower voriconazole concentrations.30 This may lead to treatment failure in patients with these genotypes. However, this has not been proven to date.
CYP2C19 genotype appears to affect the interaction of voriconazole with St. John’s wort. The baseline clearance of voriconazole and the absolute increase in clearance with St. John’s wort were smaller in carriers of one or two deficient CYP2C19*2 alleles, compared with wild-type individuals.31 These results demonstrate that long-term treatment of St. John’s wort leads to prolonged extensive reduction in voriconazole exposure, with CYP2C19 wild-type individuals being at higher risk for potential treatment failure. Similar effects on voriconazole could be seen with induction of CYP2C19 by other drugs. This could have major clinical significance as patients on voriconazole are often on other drugs at the same time.
Cytochrome P450 3A
CYP3As are the most abundant CYPs in human liver and participate in the metabolism of about 60% of drugs. Overall CYP3A4 is the most important contributor to drug oxidation, with significant contribution from CYP3A5 and CYP3A7. Important substrates in the anti-infective area include most HIV protease inhibitors and macrolide antibiotics.
Many variants have been described for CYP3A4, but their low allelic frequencies do not seem to be able to account for the high observed phenotypic variability. Genetic variation is also not well understood for CYP3A4 and haplotype analysis may be more useful. Nevertheless, the CYP3A4*1B polymorphism was found to influence the pharmacokinetics and hyperlipidemic effects of indinavir.32
Unlike CYP3A4, the hepatic expression of CYP3A5 is distributed bimodally due to a significant gene polymorphism. The active enzyme is encoded by the wild-type allele CYP3A5*1 that has a low frequency of 5–7% in Caucasians, but 40% in Africans and African Americans, and 25% in Asians.
The CYP3A5*1 genotype was associated with increased saquinavir clearance, probably from the effect of the contribution of hepatic rather than intestinal CYP3A5.33 However, the effect of CYP3A5 polymorphisms was found to be small for atazanavir34 and indinavir.35
Genetic variation in the regulatory nuclear receptors CAR and PXR that mediate induction of CYPs 3A by drugs may also contribute to antiretroviral pharmacokinetics. For example, the PXR 63396C>T (rs2472677) SNP was found to increase unboosted atazanavir clearance.36
CYP3A is induced by rifampin, NVP, EFV, dexamethasone, carbamazepine, phenytoin, and St. John’s wort that act via the PXR and CAR nuclear receptors. Rifampin and rifabutin are commonly coadministered with antiretroviral drugs because of the increased incidence of tuberculosis in HIV patients. The reduction of HIV protease inhibitor concentrations may reduce its efficacy and result in treatment failure or resistance. However, this phenomenon does not seem to be mediated by genetic factors.37 Similarly coadministering EFV or NVP with HIV protease inhibitors may reduce their efficacy. However, this effect may also be overcome by adjusting doses.38
CYP3A is inhibited by inhibitors including ketoconazole, troleandomycin, ritonavir, and bergamottin, which is the active inhibitor in grapefruit juice. Low-dose ritonavir is now used to boost other protease inhibitors. It is unclear if genetics can modify this boosting effect.
PHASE 2 ENZYMES
N-acetyltransferase Type 2 (NAT2)
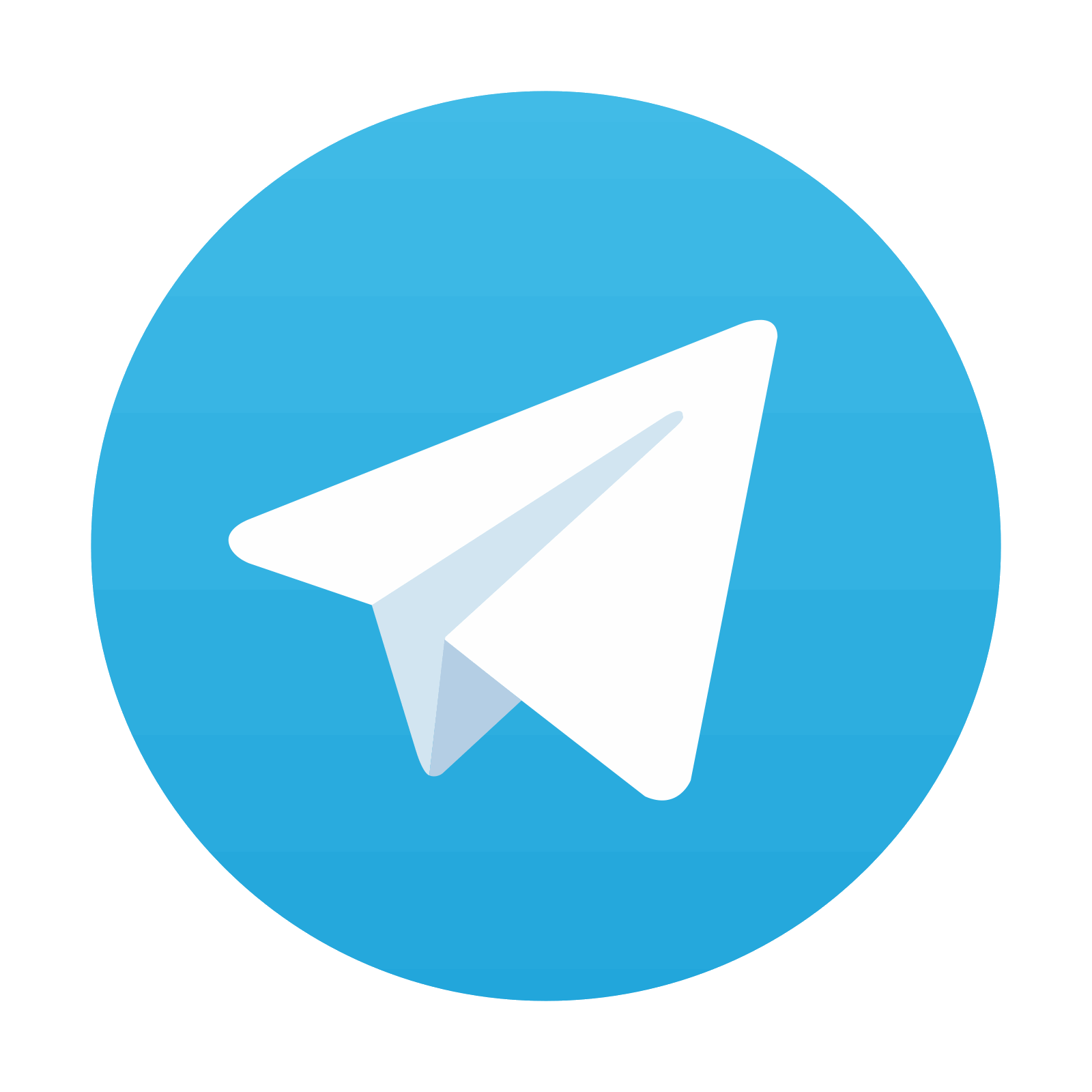
Stay updated, free articles. Join our Telegram channel
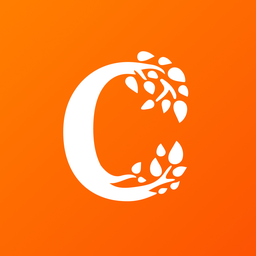
Full access? Get Clinical Tree
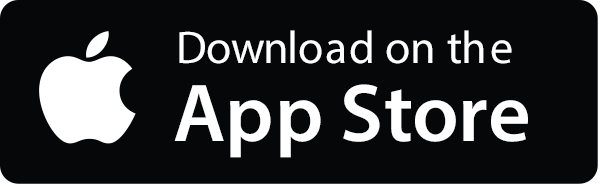
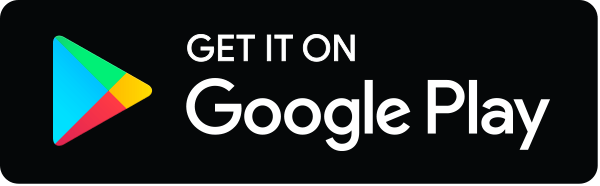