27
Pentose Phosphate Pathway and the Synthesis of Glycosides, Lactose, Glycoproteins, and Glycolipids
Glucose is used in a number of pathways other than glycolysis and glycogen synthesis. The pentose phosphate pathway (also known as the hexose monophosphate shunt, HMP shunt) consists of both oxidative and nonoxidative components (Fig. 27.1). In the oxidative pathway, glucose 6-phosphate is oxidized to ribulose 5-phosphate (ribulose 5-P), CO2, and NADPH. Ribulose 5-phosphate, a pentose, can be converted to ribose 5-phosphate (ribose 5-P) for nucleotide biosynthesis. The NADPH is used for reductive pathways, such as fatty acid biosynthesis, detoxification of drugs by monooxygenases, and the glutathione defense system against injury by reactive oxygen species (ROS).
FIGURE 27.1 Overview of the pentose phosphate pathway. The pentose phosphate pathway generates reduced nicotinamide adenine dinucleotide phosphate (NADPH) for reactions that require reducing equivalents (electrons) or ribose 5-phosphate for nucleotide biosynthesis. Glucose 6-phosphate is a substrate for both the pentose phosphate pathway and glycolysis. The five-carbon sugar intermediates of the pentose phosphate pathway are reversibly interconverted to intermediates of glycolysis. The portion of glycolysis that is not part of the pentose phosphate pathway is shown in red. ATP, adenosine triphosphate.
The sugars produced by the pentose phosphate pathway enter glycolysis as fructose 6-phosphate and glyceraldehyde 3-phosphate, and their further metabolism in the glycolytic pathway generates NADH, adenosine triphosphate (ATP), and pyruvate. The overall equation for the conversion of glucose 6-phosphate to fructose 6-phosphate and glyceraldehyde 3-phosphate through both the oxidative and nonoxidative reactions of the pentose phosphate pathway is
As had been seen previously in glycogen synthesis, several pathways for interconversion of sugars or the formation of sugar derivatives use activated sugars attached to nucleotides. Both uridine diphosphate (UDP)-glucose and UDP-galactose are used for glycosyltransferase reactions in many systems. Lactose, for example, is synthesized from UDP-galactose and glucose in the mammary gland. UDP-glucose also can be oxidized to form UDP-glucuronate, which is used to form glucuronide derivatives of bilirubin and xenobiotic compounds. Glucuronide derivatives are generally more readily excreted in urine or bile than the parent compound.
In addition to serving as fuel, carbohydrates are often found in glycoproteins (carbohydrate chains attached to proteins) and glycolipids (carbohydrate chains attached to lipids). Nucleotide sugars are used to donate sugar residues for the formation of the glycosidic bonds in both glycoproteins and glycolipids. These carbohydrate groups have many different types of functions.
Glycoproteins contain short chains of carbohydrates (oligosaccharides) that are usually branched. These oligosaccharides are generally composed of glucose, galactose, and their amino derivatives. In addition, mannose, L-fucose, and N-acetylneuraminic acid (NANA) are frequently present. The carbohydrate chains grow by the sequential addition of sugars to a serine or threonine residue of the protein. Nucleotide sugars are the precursors. Branched carbohydrate chains also may be attached to the amide nitrogen of asparagine in the protein. In this case, the chains are synthesized on dolichol phosphate and subsequently transferred to the protein. Glycoproteins are found in mucus, in the blood, in compartments within the cell (such as lysosomes), in the extracellular matrix, and embedded in the cell membrane with the carbohydrate portion extending into the extracellular space.
Many glycolipids belong to the class of sphingolipids. They are synthesized from nucleotide sugars that add monosaccharides sequentially to the hydroxymethyl group of the lipid ceramide (related to sphingosine). They often contain branches of N-acetylneuraminic acid produced from cytidine monophosphate (CMP)-NANA. They are found in the cell membrane with the carbohydrate portion extruding from the cell surface. These carbohydrates, as well as some of the carbohydrates of glycoproteins, serve as cell recognition factors.
THE WAITING ROOM 
After Al M. had been released from the hospital due to thiamin deficiency, he continued to struggle with abstaining from alcohol and injured his arm after falling down in the street while intoxicated. His friends took him to the hospital when he developed a fever of 101.5°F 2 days after hurting his arm. One of the physicians noticed that one of the lacerations on Al M.’s arm was red and swollen with some pus drainage. The pus was cultured and gram-positive cocci were found and identified as Staphylococcus aureus. Because his friends stated that he had an allergy to penicillin, and the concern over methicillin-resistant S. aureus, Al M. was started on a course of the antibiotic combination of trimethoprim and sulfamethoxazole (TMP/sulfa). To his friend’s knowledge, he had never been treated with a sulfa drug previously.
On the third day of therapy with TMP/sulfa for his infection, Al M. was slightly jaundiced. His hemoglobin level had fallen by 3.5 g/dL from its value at admission, and his urine was red-brown because of the presence of free hemoglobin. Al M. had apparently suffered acute hemolysis (lysis or destruction of some of his red blood cells) induced by his infection and exposure to the sulfa drug.
To help support herself through medical school, Edna R. works evenings in a hospital blood bank. She is responsible for assuring that compatible donor blood is available to patients who need blood transfusions. As part of her training, Edna R. has learned that the external surfaces of all blood cells contain large numbers of antigenic determinants. These determinants are often glycoproteins or glycolipids that differ from one individual to another. As a result, all blood transfusions expose the recipient to many foreign immunogens. Most of these, fortunately, do not induce antibodies, or they induce antibodies that elicit little or no immunological response. For routine blood transfusions, therefore, tests are performed only for the presence of antigens that determine whether the patient’s blood type is A, B, AB, or O, and Rh(D)-positive or Rh(D)-negative.
Jay S.’s psychomotor development has become progressively more abnormal (see Chapter 14). At 2 years of age, he is obviously severely developmentally delayed and nearly blind. His muscle weakness has progressed to the point that he cannot sit up or even crawl. As the result of a weak cough reflex, he is unable to clear his normal respiratory secretions and has had recurrent respiratory infections.
I. The Pentose Phosphate Pathway
The pentose phosphate pathway is essentially a scenic bypass route around the first stage of glycolysis that generates NADPH and ribose 5-phosphate (as well as other pentose sugars). Glucose 6-phosphate is the common precursor for both pathways. The oxidative first stage of the pentose phosphate pathway generates 2 mol of NADPH per mole of glucose 6-phosphate oxidized. The second stage of the pentose phosphate pathway generates ribose 5-P and converts unused intermediates to fructose 6-P and glyceraldehyde 3-P in the glycolytic pathway (see Fig. 27.1). All cells require NADPH for reductive detoxification, and most cells require ribose 5-P for nucleotide synthesis. Consequently, the pathway is present in all cells. The enzymes reside in the cytosol, as do the enzymes of glycolysis.
A. Oxidative Phase of the Pentose Phosphate Pathway
In the oxidative first phase of the pentose phosphate pathway, glucose 6-phosphate undergoes an oxidation and decarboxylation to a pentose sugar, ribulose 5-phosphate (Fig. 27.2). The first enzyme of this pathway, glucose 6-phosphate dehydrogenase, oxidizes the aldehyde at carbon 1 and reduces NADP+ to NADPH. The gluconolactone that is formed is rapidly hydrolyzed to 6-phosphogluconate, a sugar acid with a carboxylic acid group at carbon 1. The next oxidation step releases this carboxyl group as CO2, with the electrons being transferred to NADP+. This reaction is mechanistically very similar to the one catalyzed by isocitrate dehydrogenase in the TCA cycle. Thus, 2 mol of NADPH per mole of glucose 6-phosphate are formed from this portion of the pathway.
FIGURE 27.2 Oxidative portion of the pentose phosphate pathway. Carbon 1 of glucose 6-phosphate is oxidized to an acid and then released as CO2 in an oxidation followed by a decarboxylation reaction. Each of the oxidation steps generates a reduced nicotinamide adenine dinucleotide phosphate (NADPH).
NADPH, rather than NADH, is generally used in the cell in pathways that require the input of electrons for reductive reactions because the ratio of NADPH/NADP+ is much greater than the NADH/NAD+ ratio. The NADH generated from fuel oxidation is rapidly oxidized back to NAD+ by NADH dehydrogenase in the electron transport chain, so the level of NADH in the cell is very low.
NADPH can be generated from a number of reactions in the liver and other tissues, but not red blood cells. For example, in tissues with mitochondria, an energy-requiring transhydrogenase located near the complexes of the electron transport chain can transfer reducing equivalents from NADH to NADP+ to generate NADPH.
NADPH, however, cannot be oxidized directly by the electron transport chain, and the ratio of NADPH to NADP+ in cells is >1. The reduction potential of NADPH, therefore, can contribute to the energy needed for biosynthetic processes and provide a constant source of reducing power for detoxification reactions.
The ribulose 5-phosphate formed from the action of the two oxidative steps is isomerized to produce ribose 5-phosphate (a ketose-to-aldose conversion, similar to fructose 6-phosphate being isomerized to glucose 6-phosphate). The ribose 5-phosphate can then enter the pathway for nucleotide synthesis, if needed, or it can be converted to glycolytic intermediates, as described below for the nonoxidative phase of the pentose phosphate pathway. The pathway through which the ribose 5-phosphate travels is determined by the needs of the cell at the time of its synthesis.
B. Nonoxidative Phase of the Pentose Phosphate Pathway
The nonoxidative reactions of this pathway are reversible reactions that allow intermediates of glycolysis (specifically, glyceraldehyde 3-P and fructose 6-P) to be converted to five-carbon sugars (such as ribose 5-P), and vice versa. The needs of the cell determine in which direction this pathway proceeds. If the cell has produced ribose 5-P but does not need to synthesize nucleotides, then the ribose 5-P is converted to glycolytic intermediates. If the cell still requires NADPH, the ribose 5-P is converted back into glucose 6-P using nonoxidative reactions (see below). And finally, if the cell already has a high level of NADPH but needs to produce nucleotides, the oxidative reactions of the pentose phosphate pathway are inhibited, and the glycolytic intermediates fructose 6-P and glyceraldehyde 3-P are used to produce the five-carbon sugars using exclusively the nonoxidative phase of the pentose phosphate pathway.
1. Conversion of Ribulose 5-Phosphate to Glycolytic Intermediates
The nonoxidative portion of the pentose phosphate pathway consists of a series of rearrangement and transfer reactions that first convert ribulose 5-phosphate to ribose 5-phosphate and xylulose 5-phosphate, and then the ribose 5-phosphate and xylulose 5-phosphate are converted to intermediates of the glycolytic pathway. The enzymes involved are an epimerase, an isomerase, transketolase, and transaldolase.
The epimerase and isomerase convert ribulose 5-phosphate to two other five-carbon sugars (Fig. 27.3). The isomerase converts ribulose 5-phosphate to ribose 5-phosphate. The epimerase changes the stereochemical position of one hydroxyl group (at carbon 3), converting ribulose 5-phosphate to xylulose 5-phosphate.
FIGURE 27.3 Ribulose 5-phosphate can be epimerized (to xylulose 5-phosphate, shown in red) or isomerized (to ribose 5-phosphate, shown in the yellow box).
Transketolase transfers two-carbon fragments of keto sugars (sugars with a keto group at carbon 2) to other sugars. Transketolase picks up a two-carbon fragment from xylulose 5-phosphate by cleaving the carbon–carbon bond between the keto group and the adjacent carbon, thereby releasing glyceraldehyde 3-phosphate (Fig. 27.4). The two-carbon fragment is covalently bound to thiamin pyrophosphate, which transfers it to the aldehyde carbon of another sugar, forming a new ketose. The role of thiamin pyrophosphate here is thus very similar to its role in the oxidative decarboxylation of pyruvate and α-ketoglutarate (see Chapter 24, Section I.B). Two reactions in the pentose phosphate pathway use transketolase: In the first, the two-carbon keto fragment from xylulose 5-phosphate is transferred to ribose 5-phosphate to form sedoheptulose 7-phosphate; and in the other, a two-carbon keto fragment (usually derived from xylulose 5-phosphate) is transferred to erythrose 4-phosphate to form fructose 6-phosphate.
FIGURE 27.4 Two-carbon units transferred by transketolase. Transketolase cleaves the bond next to the keto group and transfers the two-carbon keto fragment to an aldehyde. Thiamin pyrophosphate carries the two-carbon fragment, forming a covalent bond with the carbon of the keto group.
Transaldolase transfers a three-carbon keto fragment from sedoheptulose 7-phosphate to glyceraldehyde 3-phosphate to form erythrose 4-phosphate and fructose 6-phosphate (Fig. 27.5). The aldol cleavage occurs between the two hydroxyl carbons adjacent to the keto group (on carbons 3 and 4 of the sugar). This reaction is similar to the aldolase reaction in glycolysis, and the enzyme uses an active amino group, from the side chain of lysine, to catalyze the reaction.
FIGURE 27.5 Transaldolase transfers a three-carbon fragment that contains an alcohol group next to a keto group.
The net result of the metabolism of 3 mol of ribulose 5-phosphate in the pentose phosphate pathway is the formation of 2 mol of fructose 6-phosphate and 1 mol of glyceraldehyde 3-phosphate, which then continue through the glycolytic pathway with the production of NADH, ATP, and pyruvate. Because the pentose phosphate pathway begins with glucose 6-phosphate and feeds back into the glycolytic pathway, it is sometimes called the hexose monophosphate shunt (a shunt or a pathway for glucose 6-phosphate). The reaction sequence starting from glucose 6-phosphate and involving both the oxidative and nonoxidative phases of the pathway is shown in Figure 27.6.
FIGURE 27.6 A balanced sequence of reactions in the pentose phosphate pathway. The interconversion of sugars in the pentose phosphate pathway results in the conversion of three glucose 6-phosphate to six reduced nicotinamide adenine dinucleotide phosphate (NADPH), three CO2, two fructose 6-phosphate, and one glyceraldehyde 3-phosphate.
2. Generation of Ribose 5-Phosphate from Intermediates of Glycolysis
The reactions catalyzed by the epimerase, isomerase, transketolase, and transaldolase are all reversible reactions under physiological conditions. Thus, ribose 5-phosphate required for purine and pyrimidine nucleotide synthesis can be generated from intermediates of the glycolytic pathway, as well as from the oxidative phase of the pentose phosphate pathway. The sequence of reactions that generate ribose 5-phosphate from intermediates of glycolysis is as follows:
C. Role of the Pentose Phosphate Pathway in Generation of NADPH
In general, the oxidative phase of the pentose phosphate pathway is the major source of NADPH in cells. NADPH provides the reducing equivalents for biosynthetic reactions and for oxidation–reduction reactions involved in protection against the toxicity of ROS (see Chapter 25). The glutathione-mediated defense against oxidative stress is common to all cell types (including red blood cells), and the requirement for NADPH to maintain levels of reduced glutathione probably accounts for the universal distribution of the pentose phosphate pathway among different types of cells. Figure 27.7 illustrates the importance of this pathway in maintaining the membrane integrity of the red blood cells. NADPH is also used for anabolic pathways, such as fatty acid synthesis, cholesterol synthesis, and fatty acid chain elongation (Table 27.1). It is the source of reducing equivalents for cytochrome P450 hydroxylation of aromatic compounds, steroids, alcohols, and drugs. The highest concentrations of glucose 6-phosphate dehydrogenase are found in phagocytic cells, where NADPH oxidase uses NADPH to form superoxide from molecular oxygen. The superoxide then generates hydrogen peroxide, which kills the micro-organisms taken up by the phagocytic cells (see Chapter 25).
TABLE 27.1 Pathways That Require NADPH
Detoxification |
|
|
Reductive synthesis |
|
|
|
|
|
|
FIGURE 27.7 Hemolysis caused by reactive oxygen species (ROS). (1) Maintenance of the integrity of the erythrocyte membrane depends on its ability to generate adenosine triphosphate (ATP) and reduced nicotinamide adenine dinucleotide (NADH) from glycolysis. (2) NADPH is generated by the pentose phosphate pathway. (3) NADPH is used for the reduction of oxidized glutathione (GSSG) to reduced glutathione (GSH). Glutathione is necessary for the removal of hydrogen peroxide (H2O2) and lipid peroxides generated by ROS. (4) In the erythrocytes of healthy individuals, the continuous generation of superoxide ion from the nonenzymatic oxidation of hemoglobin provides a source of ROS. The glutathione defense system is compromised by glucose 6-phosphate dehydrogenase deficiency, infections, certain drugs, and the purine glycosides of fava beans. (5) As a consequence, Heinz bodies, aggregates of cross-linked hemoglobin, form on the cell membranes and subject the cell to mechanical stress as it tries to go through small capillaries. The action of the ROS on the cell membrane as well as mechanical stress from the lack of deformability result in hemolysis. Met Hb, methemoglobin; Oxy Hb, oxyhemoglobin.
The entry of glucose 6-phosphate into the pentose phosphate pathway is controlled by the cellular concentration of NADPH. NADPH is a strong product inhibitor of glucose 6-phosphate dehydrogenase, the first enzyme of the pathway. As NADPH is oxidized in other pathways, the product inhibition of glucose 6-phosphate dehydrogenase is relieved, and the rate of the enzyme is accelerated to produce more NADPH.
In the liver, the synthesis of fatty acids from glucose is a major route of NADPH reoxidation. The synthesis of liver glucose 6-phosphate dehydrogenase, like the key enzymes of glycolysis and fatty acid synthesis, is induced by the increased insulin:glucagon ratio after a high-carbohydrate meal. A summary of the possible routes that glucose 6-P may follow using the pentose phosphate pathway is presented in Table 27.2.
TABLE 27.2 Cellular Needs Dictate the Direction of the Pentose Phosphate Pathway Reactions
CELLULAR NEED | DIRECTION OF PATHWAY |
NADPH only | Oxidative reactions produce NADPH; nonoxidative reactions convert ribulose 5-P to glucose 6-P to produce more NADPH. |
NADPH + ribose 5-P | Oxidative reactions produce NADPH and ribulose 5-P; the isomerase converts ribulose 5-P to ribose 5-P. |
Ribose 5-P only | Only the nonoxidative reactions. High NADPH inhibits glucose 6-P dehydrogenase, so transketolase and transaldolase are used to convert fructose 6-P and glyceraldehyde 3-P to ribose 5-P. |
NADPH and pyruvate | Both the oxidative and nonoxidative reactions are used. The oxidative reactions generate NADPH and ribulose 5-P. The nonoxidative reactions convert the ribulose 5-P to fructose 6-P and glyceraldehyde 3-P, and glycolysis converts these intermediates to pyruvate. |
II. Interconversions Involving Nucleotide Sugars
Activated sugars attached to nucleotides are converted to other sugars, oxidized to sugar acids, and joined to proteins, lipids, or other sugars through glycosidic bonds.
A. Reactions of UDP-Glucose
Uridine diphosphate (UDP)-glucose is an activated sugar nucleotide that is a precursor of glycogen and lactose; UDP-glucuronate and glucuronides; and the carbohydrate chains in proteoglycans, glycoproteins, and glycolipids (Fig. 27.8). Both proteoglycans and glycosaminoglycans are discussed further in Chapter 47. In the synthesis of many of the carbohydrate portions of these compounds, a sugar is transferred from the nucleotide sugar to an alcohol or other nucleophilic group to form a glycosidic bond (Fig. 27.9). The use of UDP as a leaving group in this reaction provides the energy for the formation of the new bond. The enzymes that form glycosidic bonds are sugar transferases (e.g., glycogen synthase is a glucosyltransferase). Transferases are also involved in the formation of the glycosidic bonds in bilirubin glucuronides, proteoglycans, and lactose.
FIGURE 27.8 An overview of uridine diphosphate (UDP)-glucose metabolism. The activated glucose moiety of UDP-glucose can be attached by a glycosidic bond to other sugars, as in glycogen or the sugar oligosaccharide and polysaccharide side chains of proteoglycans, glycoproteins, and glycolipids. UDP-glucose also can be oxidized to UDP-glucuronate, or epimerized to UDP-galactose, a precursor of lactose. UTP, uridine triphosphate.
FIGURE 27.9 Glycosyltransferases. These enzymes transfer sugars from nucleotide sugars to nucleophilic amino acid residues on proteins, such as the hydroxyl group of serine or the amide group of asparagine. Other transferases transfer specific sugars from a nucleotide sugar to a hydroxyl group of other sugars. The bond formed between the anomeric carbon of the sugar and the nucleophilic group of another compound is a glycosidic bond. UDP, uridine diphosphate.
B. UDP-Glucuronate: A Source of Negative Charges
One of the major routes of UDP-glucose metabolism is the formation of UDP-glucuronate, which serves as a precursor of other sugars and of glucuronides (Fig. 27.10). Glucuronate is formed by the oxidation of the alcohol on carbon 6 of glucose to an acid (through two oxidation states) by a NAD+-dependent dehydrogenase (Fig. 27.11). Glucuronate is also present in the diet and can be formed from the degradation of inositol (the sugar alcohol that forms inositol trisphosphate [IP3]), an intracellular second messenger for many hormones.
FIGURE 27.10 Metabolic routes of uridine diphosphate (UDP)-glucuronate. UDP-glucuronate is formed from UDP-glucose (shown in black). Glucuronate from UDP-glucuronate is incorporated into glycosaminoglycans (GAGs), where certain of the glucuronate residues are converted to iduronate (see Chapter 47). UDP-glucuronate is a precursor of UDP-xylose, another sugar residue incorporated into glycosaminoglycans. Glucuronate is also transferred to the carboxyl groups of bilirubin or the alcohol groups of steroids, drugs, and xenobiotics to form glucuronides. The “-ide” in the name glucuronide denotes that these compounds are glycosides. Xenobiotics are pharmacologically, endocrinologically, or toxicologically active substances not produced endogenously and, therefore, are foreign to an organism. Drugs are an example of xenobiotics.
FIGURE 27.11 Formation of glucuronate and glucuronides. A glycosidic bond is formed between the anomeric hydroxyl of glucuronate (at carbon 1) and the hydroxyl group of a nonpolar compound. The negatively charged carboxyl group of the glucuronate increases the water solubility and allows otherwise nonpolar compounds to be excreted in the urine or bile. The hydrogen atoms have been omitted from the figure for clarity. UDP, uridine diphosphate.
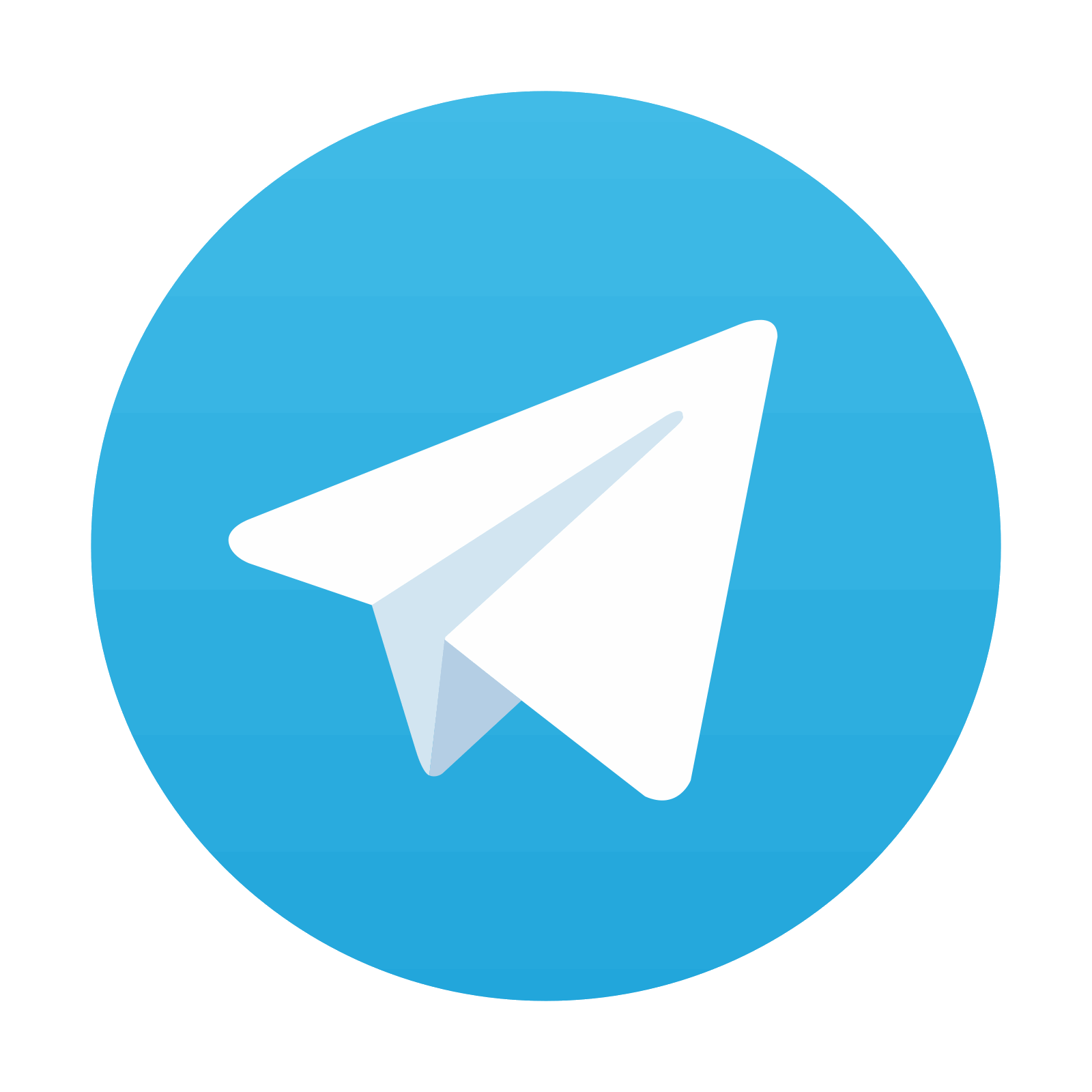
Stay updated, free articles. Join our Telegram channel
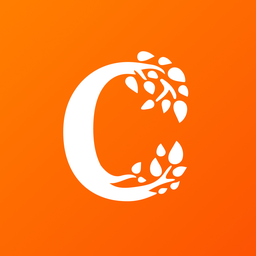
Full access? Get Clinical Tree
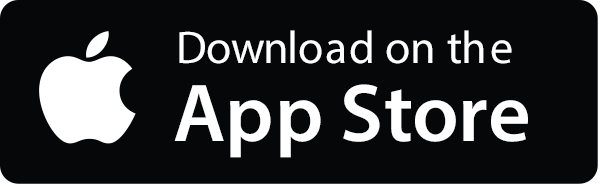
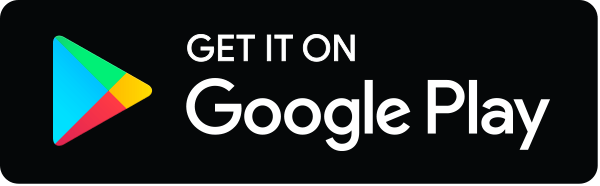