1 Gleb E. Yakubov1,2, Hannah Gibbins3, Gordon B. Proctor3 and Guy H. Carpenter3 1School of Chemical Engineering The University of Queensland Australia 2Australian Research Council Centre of Excellence in Plant Cell Walls The University of Queensland Australia 3Salivary Research Unit King’s College London Dental Institute UK Like no other mucosae, the oral cavity comprises the widest range of different tissues and types of mucosal linings. The oral cavity comprises soft oral tissues of gums, buccal surfaces, hard palate, the tongue, and lips. Teeth are by contrast made of biomineralised material with outer enamel containing up to 96% hydroxyapatite, with water and protein accounting for the remaining 4%. Such diversity stems from the multiple physiological functions of the mouth and environmental stresses that it is subject to. Temperature variations, mechanical action, food processing, defence against microorganisms and toxins (e.g. nicotine) are some of those environmental conditions that oral surfaces cope with to provide key physiological functions, such as the digestive, sensing, protective and barrier functions of the underlying tissues, pathogen resistance and immunity. The epithelium of the mouth varies considerably. In areas of high abrasion, such as the hard palate and the tongue, the top layer of epithelial cells is highly keratinised and the rete processes that hold the lamina propria to the epithelium are more apparent (Figure 1.1). In other areas, such as the cheek and under the tongue, the epithelium is not so keratinised. Oral epithelium is comprised of tightly packed layers of epithelial cells originating from the basal layer. As cells proliferate from the basal layer they start to differentiate into larger flattened squamous epithelial cells. As part of the differentiation process cells increase levels of intracellular transglutaminase. This enzyme helps to cross-link proteins within the cell into the cell wall, forming a tough proteinaceous coat that is impermeable to water and osmotic changes. Under the basal layer of epithelial cells is the lamina propria containing a rich capillary bed and fibroblasts forming the connective tissue (collagen). Depending on the location, function and proximity to the external environmental, the mechanical strength and permeability of oral mucosa may exhibit considerable variation. This variation is typically achieved through the level of keratinisation within the epithelium. The keratinised tissues (i.e. masticatory mucosa) are relatively tough, for example the hard palate and gums where the granular layer is enriched with keratin filaments. The nonkeratinised tissues (i.e. mucosal linings) are softer and more permeable, for example the floor of the mouth and buccal (i.e. cheek) surfaces. The tongue is an example of specialised mucosa; it consists of both keratinised and nonkeratinised regions. The raised bumps seen on the tip of the tongue are keratinised and have occasional taste buds. However, most taste buds are present further back on the tongue within the circumvallate papilla. The permeability of oral mucosa depends on the level of keratinisation, thickness and lipid content. The lipid content of keratinised oral tissues has some distinctive patterns compared to skin. The hard palate epidermis contains about 10-fold lower levels of cholesteryl esters and linoleate-rich acylceramide (CER1), and a 10-fold higher level of triacylglycerols. The palate epidermis also contains some significant amounts of phospholypids, such as sphingomyelin, phosphatedylcholine and phosphatedylserine, that are totally absent in the skin epidermis. Despite being less permeable compared to nonkeratinised linings, keratinised oral tissues are still some 10 times more permeable than skin due to lipid composition and the level of hydration. The thickness of the epithelium in the oral mucosa also varies, with the buccal mucosa having a 580 ± 90 μm thick epithelium compared to a 190 ± 40 μm thin epithelium of the floor of mouth (for comparison the thickness of typical skin epidermis is in the range between 100 and 120 μm). For mucoadhesive applications it is also important to consider that turnover rate is higher for nonkeratinised tissues than for keratinised ones [1–6]. Figure 1.1 Section of human hard palate mucosa stained by haematoxylin and eosin. The purple-stained epithelium overlies the pink lamina propria and the submucosal layer. (Image courtesy of Prof Peter Morgan, King’s College London, UK.) Many mucosal surfaces act as an ecological niche for microorganisms, and oral cavity is not an exception. In fact, it hosts a unique and complex microbial ecosystem, with up to 10 000 microbial species belonging to firmicutes, bacteroidetes, proteobacteria and actinobacteria phyla in the ratio approximately 40 : 30 : 20 : 5, with the remaining ∼5% being other bacterial phyla, candida fungi and some protozoa. Bacterial species are represented by both aerobic and anaerobic species (e.g. Fusobacterium nucleatum), with survival of the latter depending on their association with aerobic species [7]. The current review focuses on the structure and function of mucosa on soft oral tissues, since soft surfaces are key targets for oral transmucosal drug carriers. For a comprehensive review on oral microbiology the reader is referred to a book by Marsh and Martin, [8], and for a more detailed account of tooth surfaces and salivary tooth pellicle to a recent edition of ‘Oral Biology’ by Berkovitz [9]. The mouth is richly innervated mostly by sensory nerves although some autonomic efferents innervate the blood vessels and the minor salivary glands. The sensory nerves innervate the mucosa to detect touch, temperature, damage and tastes. The facial nerve (cranial nerve (CN VII), the glossopharyngeal nerve (CN IX), and the trigeminal nerve (CN V) innervate the oral cavity [10]. Taste buds in the posterior one-third of the tongue receive innervation from the glossopharyngeal nerve, while those in the anterior two-thirds receive innervation from the chorda tympani branch of the facial nerve [11]. Specifically, chorda tympani fibres innervate fungiform papillae and the facial nerve fibres serve the foliate and circumvallate papillae [12]. Divisions of the mandibular branch of the trigeminal nerve, namely the lingual nerves, also project to the anterior portion of the tongue, providing somatosensory innervation [13–16]. Not only do these fibres innervate the epithelia surrounding the taste buds but they have also been shown to enter fungiform papillae, forming tight bundles which are referred to as Ruffini or Meissner’s endings [17]. This end structure may be specialised for detection of touch and is more often found in the anterior part of the tongue. These mechanoreceptors (MRs) are classified according to the size and character of their receptive field [18,19]; type I MRs have small and distinct receptive fields, while type II have large, diffuse receptive fields. MRs are further classified depending on whether they are rapidly adapting (RA) or slowly adapting (SA) receptors; RA receptors respond during the dynamic phase of stimulus application and SA receptors respond to both dynamic and static force applications [20]. The distribution of MR types varies with oral cavity location. For example, recording from the infraorbital nerve, Johansson et al. [21,22] found that about one-third of the MRs at the transitional zone of the upper lip were SA I (slow adapting, type I), while Trulsson and Essick [13], recording from the lingual nerve, found that two-thirds of the MRs stimulated in the lingual mucosa were RA. They suggested that mucosal regions that are deformed during normal functioning (e.g. lips) have a greater proportion of SA afferents, while regions that are mainly used for used for explorative and manipulative behaviours (e.g. tongue) contain a proportionately greater number of RA fibres. The mandibular and infraorbital nerves provide innervation to the mucus membranes of the lower lip and cheeks, and the upper lip and cheeks, respectively, mostly as free-nerve endings sometimes associated with Merkel cells. Merkel cells are under-studied cells that lie within the lamina propria and may cause the nerves to fire in response to touch, although the exact relationship is unclear. The territory innervated by the trigeminal nerve extends to include the teeth, periodontium and the bulk of both the soft and hard palates [23]. All of these nerves – infraorbital nerve, chorda tympani, lingual nerve, glossopharyngeal nerve – contain afferent mechanoreceptive fibres. The mammalian tongue has three structures with which taste buds are associated: circumvallate, foliate and fungiform papillae (Figure 1.2). Figure 1.2 The location of the taste buds on the tongue occur in three main areas, associated with the circumvallate, foliate and fungiform papillae, which are small areas or keratinised epithelium often appearing as red dots. The taste buds in the foliate and circumvallate papillae are located with the crypts, which are constantly bathed by von Ebner’s glands – serous minor salivary glands. Adapted from [24]. Copyright © 2006, Rights Managed by Nature Publishing Group. Polarised, neuroepithelial taste receptor cells (TRCs) form clusters of 50–150 cells as taste buds, which resemble onions when sectioned histologically (Figure 1.3). The apical surface of the taste bud is exposed to the oral cavity through the taste pore, where the microvilli of TRCs make contact with saliva and tastants [25]. Interestingly, TRCs are not static receptor structures. As first demonstrated in the rat, TRCs undergo a progression from basal cells, which are the precursor cell population, through differentiation and death that ranges from two days to three weeks [26]. TRCs themselves are not neurons; they synapse onto the primary gustatory fibres of the nerves that innervate them, with each gustatory fibre contacting multiple TRCs in multiple taste buds [12]. Figure 1.3 Section of human tongue showing ‘onion-like’ taste buds (arrow) within the crypts of circumvallate papillae located towards the back of the tongue. Picture courtesy of Prof Peter Morgan, King’s College London, UK. Progress has been made in characterising the different channels responsible for the detection of the basic tastes by taste bud cells [24]. Salt tastes are transmitted by sodium and, possibly, potassium channels located on the apical surface of taste bud cells and signal to afferent nerves via ATP molecules whereas sour taste (which are protons) is detected by a separate channel [27]. Receptors for bitter tastes and glutamate have also been determined [28]. An area of intense research is the characterisation of a receptor for fatty tastes. A suitable candidate has been found in mice (CD36), however its presence and functionality have yet to be proven in humans. In addition to the basic tastes, receptors for other tastants are also being revealed. For example, oral sensation is markedly affected by activation of TRP (Transient Receptor Potential) channels present on nerve endings innervating the mucosal epithelial surfaces. TRPP(polycystic) 3 or PKD1L3 is a proton sensor that is expressed on taste receptors and mediates sour taste [29]. In contrast, TRPM(melastatin) 5, expressed on taste receptor cells in association with T1R 1,2 and 3 receptors and T2R receptors, is a downstream signalling component that appears to also account for a temperature dependent modification of the sweet, bitter and umami taste perceptions [30]. TRPV1, 3, 4, TRPM8 and TPRA1 are temperature activated channels that are also expressed on oral keratinocytes [31]. TRPV3 can activate sensory neurons through release of ATP and interaction with purinergic P2 receptors [32]. The sensation of cold in the mouth appears to evoke a flow of saliva [33] and can increase salivation in response to liquid gustatory stimulation [34]. There have been few published studies of the effects of TRP activation on salivary secretion although capsaicin, a TRPV1 channel activator, and hydroxyl-alpha-sanshool, an activator of TRPV1 and TRPA1 channels, can evoked salivary secretion [35]; there is evidence that direct activation of TRPV1 receptors expressed in salivary glands may evoke a secretory response. There are three pairs of major salivary glands: parotid, submandibular and sublingual. The parotid is located near to the ear and can sometimes be felt when blowing up balloons. Although situated near the ear, Stenson’s duct conveys the serous watery saliva adjacent to the upper third molar, sometimes apparent as fleshy papillae on the inside of the cheek. The submandibular is located near the jaw line whereas the sublingual is located under the tongue. Each salivary gland is connected to the oral cavity via a duct. However, in some people the duct from the submandibular can fuse with the ducts coming from the sublingual, so that collecting from each separately can be difficult. The salivary glands are under collaborative parasympathetic (acetylcholine) and sympathetic (noradrenaline) control via the efferent (secreto-motor) fibres of the facial and glossopharyngeal nerves [36,37]. Inside the glands, the secretion of fluid is initiated by Ca2+ signals acting on Ca2+ dependent K+ and Cl− channels. The opening of these channels facilitates the osmotic drainage of water into the lumen following the flux of Cl− ions [38]. The majority of saliva is secreted by the parotid, submandibular and sublingual exocrine glands [39]. At rest, the submandibular glands contribute the majority whereas during stimulation by taste or chewing the parotid is the major secretor [40]. It is through these glands that salivary proteins and enzymes are secreted into the oral cavity, where they provide lubrication and initiate the process of digestion [41]. Soft tissues of oral mucosa host small (1–2 mm) topical secretory apparatuses, called minor salivary glands. These are distributed throughout the oral cavity, with some notable locations in the tissues of the buccal, labial and lingual mucosa. Innervated by the VII cranial nerve, minor salivary glands contribute only about 10% to the total volume of human saliva released into the oral cavity [42,43]. Despite the small volume of secretions, minor glands produce mucin- and immunoglobulin-rich saliva; according to Siqueira et al., at least eight different immunoglobulins can be identified in labial minor gland secretions [44]. This has a significant contribution to the maintenance of oral health, also due to their proximity to mucosal surfaces [45]. A notable exception are the von Ebner’s glands (also called gustatory glands), which are located proximally to the circumvallate and foliate papillae in the tongue. Their secretion is serous, which facilitates the transport of tastant molecules to the taste buds, and hence participates in taste perception. On a typical mucosal tissue, the mucus lining is synthesised by specialised mucus-producing (goblet) epithelial and submucosal cells. Oral mucosa is different, however; most of the proteinaceous components covering the mucosa are synthesised outside the oral cavity. Saliva synthesis occurs in salivary glands; it is then excreted into the oral cavity through salivary ducts. Upon excretion, glandular saliva secretions are mixed, and proteins and mucins self-assemble to form the salivary film that acts as a lining of oral mucosa. The secretion of saliva is continuous, and the estimates suggest that, on average, an adult consumes up to half a litre of his or her own saliva a day. This constant flow leads to a saliva turnover rate of about 10 minutes. In resting, salivary flow is anywhere between 0.1 and 0.5 ml/min whereas upon stimulation the rate increases up to 1–5 ml/min and varies highly between individuals. At rest, the submandibular glands contribute 69%, the parotid 26%, and the sublingual contributes 5% to the total secretions [46]. Saliva secretion can be stimulated by mastication and some gustatory stimuli, such as acids and, to a lesser extent, bitter and umami tastants. Contrary to intuitive viewpoints, sweet tastants have the lowest propensity to inducing saliva production, which from an evolutionary point of view may be associated with the high aqueous solubility of carbohydrates. Depending on stimulation, different glands yield different reactions. Chewing and other mechanical actions stimulate primarily parotid secretions that are relatively serous and have low viscoelasticity. Such rheological properties are advantageous for food bolus formation and aid swallowing. An important factor affecting transmucosal transport of ionic substances (e.g. organic salts) is the ionic composition of saliva. Although primary saliva is formed by osmotic gradients and would, therefore, taste salty (like sweat for example), special cells within the salivary gland reabsorb most of the salt to create saliva that is hypotonic with respect to blood. At rest, the concentration of potassium ions exceeds that of sodium ions, 15–25 mmol/l (K+) versus 1–3 mmol/l (Na+). However, upon stimulation the glands are not able to reabsorb as much salt, which reverses this ratio, so that sodium concentration increases up to 35 mmol/l and, in some instances, even up to 100 mmol/l. At the same time, potassium levels stay at approximately the same level as in resting saliva [46,47]. The buffer capacity of saliva is maintained mostly by bicarbonate buffer, which plays a role in maintaining salivary pH around 6.5–7, which in turn ensures ion equilibrium is acting to effect dental remineralisation. Factors influencing salivary flow rate tends to decrease its buffer capacity and to increase the risk of developing xerostemia and caries [48–50]. Salivary proteome comprises more than a thousand protein species [51]. Whole mouth saliva contains both proteins synthesised in the glands as well as some traces of components infiltrated from blood that enter the mouth via the gingival margins surrounding teeth. There are six major classes of salivary proteins/glycoproteins: mucins (represented by MUC5B and MUC7 genetic types); acidic, basic and heavily glycosylated proline-rich proteins; salivary amylases; statherins; histatins; and cystatins. In addition, saliva contains significant amounts of salivary immunoglobulin A, carbonic anhydrase, lactoferrin, lysozyme, lactoperoxidase and serum albumin. Each gland produces a different set of proteins. According to the proteome analysis by Denny et al. [51], out of 1116 identifications 665 were found in both parotid and sublingual–submandibular (SLSM) glandular secretions, while 249 and 252 identifications were specific to parotid and sublingual–submandibular secretions, respectively. About 24–26% of proteins in saliva are shared with tears and about 19% with blood. Although glandular saliva secretions typically contain representatives from all major classes, there are notable exceptions and deviations (especially if amounts of secreted proteins are taken into consideration). For example, the majority of salivary α-amylase is secreted from parotid glands, while gelling MUC5B and soluble MUC7 mucins originate from SMSL secretions. Most salivary proteins cannot be found anywhere else, hence only 19% of proteins are shared with, for example, plasma proteome. The majority of salivary proteins are glycosylated [52] and/or phosphorylated [53]. These peculiar properties are behind the reason that salivary proteins are participating in the various heterotypic complexes with an intricate pattern of protein–protein interaction. Progress in protein/peptide screening and identification opened up a number of opportunities for more detailed accounts of salivary proteome [54–56]. Within this, the major focus is on identifying markers for oral health [57], obesity [58], salivary gland diseases and cancers [59,60] that can be tested using salivary diagnostics [61]. Mucins are ubiquitous glycoproteins and can be found in all metazoan species [62]. They form a glycocalyx layer around all animal cells and are a key component of mucus in all mucosal tissues [63]. Salivary mucins are represented by two genetic types, MUC5B and MUC7. Based on gel electrophoresis data, salivary mucin fractions appear in two spots; MG1 (high molecular weight >1 MDa) and MG2 (in the range 100–300 kDa). The MG1 fraction is primarily comprised of different glycoforms of MUC5B mucins [64], while the MG2 fraction is primarily MUC7 mucins. Each genetic type is represented by a number of glycoforms. The majority of glycosylation is via O-links, which are when oligosaccharide chains are attached to hydroxyl groups of serine or threonine residues via the N-acetylgalactosamine residue of an oligosaccharide chain. There is a small number of N-glycosidic links formed between asparagine and N-acetylglucosamine [65]. The pattern of mucin glycosylation is very diverse; many oligosaccharide side chains are negatively charged due to terminal sialic acid [63]. A considerable fraction of MUC5B mucins secreted from minor salivary glands have an oligosaccharide structure containing terminal sulfonated saccharide residues with pKa less than 2 [66]. The general structure of the MUC5B single unit comprises about 5000 amino acids and has a structure of a tri-block. The N terminus comprises of several von Willebrand factor type D-domains that contain a number of cysteine residues as well as charged amino acids. In contrast MUC7 is shorter and does not contain von Williebrand factor regions and is thus thought not to contribute to gel formation. The C terminus of MUC5B comprises D-domains and cysteine-knot domains. Both termini are largely nonglycosylated and rich in cysteine residues, which makes them form disulfide bridges [67,68]. In between the terminal blocks, there is a long tandem repeat region that is rich in serine and threonine and densely decorated with a ‘bottle brush’ of O-linked oligosaccharide side chains [68]. The peculiarity of MUC5B tandem repeat region is that it comprises 3570 amino acids arranged in four repeats interrupted by cysteine-rich subdomains. The interruption in glycosylation renders the heavily glycosylate middle block less rigid compared to other mucins, such as MUC2 (intestinal mucin). Due to lower rigidity MUC5B tends to form weaker gels, which is instrumental for saliva to remain fluid. Due to the tri-block nature of mucins, with terminal blocks being nonglycosylated, mucins adopt a dumb-bell conformation in the solution [69–71], whereby D-domains are folded in a coiled globule with the size of about 5–20 nm. The length of the glycosylation part varies considerably depending on the mucin type and post-transcriptional splicing and there are differences in the glycosylation of MUC5B and MUC7. Overall, most of physicochemical properties of mucins depend on glycosylation and their molecular weight. The MUC5B mucins, glycosylation of which is particularly heterogeneous, can be roughly split in two large clusters depending on their charge: neutral and charged. These two clusters roughly correspond with two fractions of MUC5B that can be obtained using ultracentrifugation: the gel fraction and the sol fraction [66,72,73]. There is evidence that charged mucins with a higher content of sialic acid are more common in the sol fraction, as their stability is promoted by the electrostatic nature of sialic acid. By contrast, more neutral mucins tend to assemble in larger oligomeric structures [68]. The investigation of friction between saliva-modified surfaces revealed that it is this sol fraction that plays an important role in forming an adsorbed salivary film and resulting in low friction response. The gel fraction, containing supramolecular aggregates, is, on the other hand, responsible for saliva’s viscoelastic rheological behaviour. The formation of mucin gel occurs in conjunction with calcium cross-linking [74,75], disulfide bridging, hydrophobic forces and interactions with proline-rich proteins (PRPs) and other lower molecular weight salivary proteins (e.g. sIgA, lysozyme, histatins) [76,77]. Mucin polymerisation may happen before full secretion and post-translational glycosylation [78,79]. Except for disulfide bridges between cysteine residues, all other interactions are noncovalent. The location of cysteine residues at terminal areas of D-domains is instrumental in head-to-tail mucin aggregation, as well as in adsorption to substrates [80]. Due to multiple types of interactions, mucins form a dynamic network with complex chain topology. Depending on the topological association, mucin molecules can also form higher-order assemblies [74,75]. The effect of Ca2+ involves both nonglycosylated units as well as oligosaccharide residues terminated with negatively charged sialic acid [81]. Thus, mucin assemblies can involve stacking of glycosylated ‘bottle brushes’ that leads to the emergence of nematic order in concentrated solutions [82]. Hydrophobic interaction plays important role in sol–gel transition, as well as in adsorption of mucin on surfaces [83]. The dynamic network of mucins and their ability to bind to various chemistries (including hydrophobic groups) is instrumental for transport properties of saliva and salivary film. On one hand, the mucin network creates a pore size distribution that is of the order of 100–200 nm for the pellicle [84,85], which is important for transport of nanocarriers. It is important to note that this size pore is strongly dependent on mucin concentration, ionic strength and so on. [86]. This model can prove useful for future studies of the transport properties of nanoparticles through mucus layers, which may provide a new toolbox for designing new methods of drug delivery across the mucosal films [83]. On another hand, mucin biopolymers may offer competing binding sites that trap viruses inside the biopolymer matrix. As a consequence, those viruses are prevented from reaching the epithelial surface between the mucin sugar groups and the virus capsids might be responsible for the trapping of the virus particles. Which combination of physical forces regulates these binding interactions and how they depend on the detailed buffer milieu is a complex question that will need to be addressed in detail in future experiments [87]. Proline-rich proteins are a broad class of unstructured naturally unfolded proteins [88,89] that account for nearly 70% of proteinaceous components in parotid saliva, with the major groups being acidic, basic and glycosylated PRPs (reviews can be found elsewhere [90–93]). Most of the basic PRPs are secreted by the parotid glands, with concentrations increasing up to 50% upon stimulation. The average whole saliva content of PRPs (resting conditions) varies between individuals and is about 430 ± 125 μg/ml [94]. It is also evident that PRPs participate in aggregation of salivary proteins, with approximately 10–25% of PRPs being lost upon saliva centrifugation [94]. Due to a highly segregated distribution of charged amino acids, PRPs adopt extended conformation with flexible structure, thus bearing some structural resemblance to milk caseins [95,96]. This structural property affects PRPs’ ability to adsorb on a variety of surface chemistries; studies have shown that PRPs indeed exhibit a high film forming capacity on both hydrophilic and hydrophobic surfaces [97]. About one-third of the PRPs are acidic and appear to have functions associated with mineral homoeostasis of tooth enamel [98]. Acting together with statherins, acidic PRPs act as inhibitors of spontaneous precipitation of calcium phosphate (CaPO4) salts and prevent secondary crystal growth by adsorbing on the enamel. Basic PRPs are minor constituents of dental pellicle [99], with their role being associated with binding onto the bacterial proteoglycan cell walls. Thus, it has been shown that PRPs display selective binding to Streptococcus mutans and Actinomyces viscosus [100,101] Statherin’s polypeptide chain consists of 43 amino acids (Mw ∼5.4 kDa). Immunogold staining technique inside the granules of serous cells were used to demonstrate its presence in secretions of both parotid and submandibular glands, with a much weaker presence in major sublingual glands [102]. Statherin is rich in tyrosine and has two phosphorylation sites on serine 2 and 3. Statherin has high degree of charge and structural asymmetry. Ten of the twelve charged groups occur in the N-terminal that itself consists of only 13 residues. The N-terminal also features an exceptional grouping of five negatively charged residues that form a core of the Ca2+ binding domain. The tyrosine, proline and glutamine residues are confined to the carboxyl terminal that spans two-thirds of the statherin molecule. These three amino acids account for 75% of the residues present in this segment, resulting in a 310 helix structure being featured in the C-terminal between the residues Pro36 and Phe43. In the central part, a polyproline type II helix is formed in the region between residues Gly19 and Gln35 [103,104], thus forming a characteristic kink in the statherin that makes it resemble a short-footed Latin letter ‘L’. The investigation of statherin conformation upon adsorption revealed the significant changes occurring with the C-terminal region. Upon adsorption onto the hydroxyapatite crystals, it re-folds into an α-helix. This folded pattern can be recognised by antibodies, as was shown from analysis of binding of oral pathogens that selectively recognise hydroxyapatite-bound statherin [105–108]. Furthermore, binding onto hydroxyapatite greatly enhanced its lubricating qualities [109]. Unlike most salivary proteins, statherin (partly due to its small size) has been extensively investigated using recombinant proteins [110,111]. A number of studies tested statherin fragments to investigate the role of each of the statherin subunits in its functionality [112,113]. It was established that the negatively charged N-terminal domain is responsible for specific adsorption of statherin on the hydroxyapatite mineral surface, while in the bulk salivary film this domain forms a coordination complex with Ca2+ ions. This dual functionality therefore inhibits both the spontaneous (or primary) and crystal growth (or secondary) mechanisms of calcium phosphate precipitation from saliva. Due to very strong adsorption on the enamel surfaces, statherin is one of the major precursor of the enamel pellicle. Since the C-terminal of statherin is uncharged and the N-terminal is strongly charged, it often acts as a surfactant. This surfactant functionality is responsible for saliva surface tension and interfacial elasticity [114]. It also contributes to the surface wetting (i.e. Marangoni flow) and promoting lubrication through facilitation of salivary film entrainment into the contact between oral surfaces. Surfactant properties are also an additional factor participating in the mechanism of hydroxyapatite crystal growth inhibition, which is due to formation of a structured adsorbed pellicle that forms an additional energy barrier preventing Ca2+ adsorption. Statherin was also found to bind strongly to hydrophobic surfaces (through the C-domain) [97,115], which also explains its ability to bind to bacterial surfaces, such as fimbriae that mediate bacterial cell adhesion to the surface of the tooth [96]. Cystatins are a group of inhibitors of cysteine proteinases that are a class of proteolytic enzymes found in all living species [116]. With respect to the human oral environment, some substantial quantities of cysteine proteases can come with food, for example, ficin (form figs) and papain (from pineapples). In saliva, the majority of cystatins are represented by cystatin S (phosphorilated), cystatin SN (neutral pI) and cystatins SA (acidic pI). The phosphorylated cystatin S exists primarily in two forms: mono- and diphosphorylated [117–123]. Importantly, the diphosphorylated form was not detected anywhere else but saliva, suggesting that Ca2+ binding motif is an evolutionary selection factor. Each of the proteins is encoded by its respective gene but all contain 121 amino acids and showed 90% sequence homology with one another [124]. In addition to cystatins S/SA/SN, saliva contains a detectable amount of cystatins C and D, which belong to another family of cystatins and bear about 60% homology with S-types. These proteins can have an important role in inflammation and pathogen response, and are under the scrutiny as prognostic markers for several forms of cancer [125–127]. Cystatins are primarily synthesised in submandibular glands with minor quantities coming with the parotid secretions [128]. The primary function of cystatins is protease inhibition and, hence, response to inflammation, dental plaque activity, as well as antiviral activity. A particular activity has been reported against Herpes simplex virus type 1 [129]. Salivary cystatins are also involved in the formation of the enamel pellicle acquired in vivo, as can be inferred from the presence of diphosphorylated forms that play a role in enamel mineralisation processes [130–132]. Histatins are a group of small histidine-rich salivary proteins (often classified as peptides). Unlike many salivary proteins that can be found in saliva secretions of other animals or at least share similarity across mammals, histatins appear to be quite specific to humans and some nonhuman primates [133]. There are two genetic types of human histatins, HIS 1 and HIS 2, that code histatin 1 (38 amino acids) and histatin 3 (32 amino acids), respectively. A 24 amino acid long histatin 5 is a hydrolysis product of histatin 3. The concentration of histatins in whole saliva is rather low, about 33 ± 17 μg/ml, with histatin 1 being the most abundant type, accounting for about 60% of all whole mouth histatins. The concentration of histatins in parotid secretions is at least fivefold higher than in whole mouth saliva, thus suggesting their rapid adsorptive interactions with oral surfaces, digestion and/or dilution with submandibular secretions [94]. The primary role of histatins is in nonimmune defence response. A strong antimicrobial activity was documented against Candida Albicans (including the activity towards blastospores), Criptococcus spp. and Streptococcus mutans [134]. The majority of the antimicrobial activity assay reports favoured the shortest histatin 5 peptide as the one with highest antimicrobial effect [135–137]. Due to histatins’ activity a number of leads have been suggested for a new generation of antimicrobial compounds for the treatment of oral mycoses. In addition, an important role of histatins is that they participate in wound healing [138]. It is known that wounds in the mouth heal faster and with less scarification and inflammation than those in the skin. It was also reported that histatins enhance epithelium growth by inducing cell spreading and migration, which establishes the experimental basis for the development of synthetic histatin-like peptides as novel skin wound healing agents. [139,140]. Another factor that is crucial for oral processing of food and beverages is binding with dietary tannins. Histatins are not the only proteins in saliva to bind tannins. Proline-rich proteins remain the major factors; however, at neutral pH histatin 5 was found to be the most effective precipitant of both condensed tannin and tannic acid [141]. Finally, histatins are a prominent component of salivary pellicle on both enamel and soft oral tissues. Salivary amylase is a key starch digesting enzyme acting during oral processing of starch-containing foods, and later in the stomach environment before it deactivates due to the low pH of the stomach environment. Unlike previously thought, the pH of the interior of the bolus remains roughly neutral for some 20–30 minutes inside the stomach. Thereafter, pH decreases to a low enough level to deactivate salivary amylase [142]. Hence, starch pre-processing may greatly increase the efficiency of digestion of starch-based foods, as well as direct food preferences, due to the release of sweet tasting low molecular weight carbohydrates (e.g. glucose) [143]. It was found that the number of copies of salivary amylase genes correlates positively with salivary amylase protein level and that individuals from populations with high-starch diets have, on average, more AMY1 copies than those with traditionally low-starch diets, thus suggesting the adaptation mechanism being in place [141]. However, person-to-person variations in amylase levels do not correlate to a subject’s liking for starchy foods. The self-assembly process of salivary proteins on oral tissues leads to formation of different types of salivary film on different oral mucosal surfaces. The properties of salivary film depend on the substrate and its relative proximity to the glands. It was found that the thickness of salivary film measured using fluid soaking paper strips spans from 10 μm on the anterior hard palate up to about 50 μm at the anterior of the tongue, with buccal surfaces having intermediate values of thickness. The protein content of these salivary films also varies considerably, as shown in Table 1.1 [144]. A notable variation in protein content is clearly observable across oral surfaces, with the hard palate and lower labial surfaces having a protein content at least seven times higher than whole mouth saliva and to that of the salivary film on the tongue. Table 1.1 Calculated thickness and protein concentration of residual mucosal fluids collected from healthy volunteers. Adapted from Pramanik et al. [144] The variation in distribution of salivary pellicle thickness and its protein content translates into a broader context and can be used to characterise dry-mouth conditions. In dry-mouth patients, who display flow rates below 0.1 mg/ml, the salivary films across different areas are roughly twofold thinner, with up to a 10-fold higher protein content (Table 1.2). This may have significant implications to flow of saliva and formation of hydrated salivary pellicle. In such concentrated solutions, proteins may deposit excessively due to unspecific interaction, thus rendering hydration salivary pellicle and its lubrication properties. Table 1.2 Calculated thickness and protein concentration of residual mucosal fluids collected from dry-mouth patients and controls. (Adapted from Pramanik et al. [144].) Age matched control UWMS flow ≥ 0.2 ml/min Dry-mouth patient UWMS flow ≤ 0.1 ml/min Exposure to mechanical action also contributes to the inhomogeneous properties of salivary films, as has been shown on the enamel substrates exposed to the different tooth brushing conditions [145,146]. This understanding is readily translatable to the case of soft surfaces, where abrasive action can alter the surface assembly and deposition kinetics of salivary proteins, thereby disrupting ordinary replenishment of salivary films. Saliva has the ability to form a bound proteinaceous layer on all soft tissues within the oral cavity, known as the salivary mucosal pellicle [147]. The salivary pellicle plays a key role in the maintenance of oral health by providing lubrication, hydration, immune response, shaping the microbial flora population and regulating the tooth mineralisation processes. This layer also provides a physical barrier of protection, preventing abrasion between oral surfaces, and contributes to maintaining the normal mouth feel [148,149]. All these properties make salivary film key in mediating transport of molecules, including pharmaceuticals, through oral mucosa. In the last 15 years research on the formation of salivary pellicle has attracted significant effort. The analysis of ex vivo pellicle has yielded a number of fundamental insights into the structure and the role of salivary proteins within the pellicle. Human whole saliva, single-salivary gland secretions, salivary fractions, isolated and purified salivary proteins as well as model molecules were used to examine the structure of the salivary pellicle. Many studies aimed at understanding salivary pellicle on teeth and used both enamel samples and model hydroxyapatite substrates. Later, this research was extended to include investigations of salivary pellicle formed on soft surfaces, including buccal cells. Specifically, progress has been made in understanding the effects of mucin type, mucin preparations, ambient conditions and surface properties on adsorption and assembly of salivary mixed layers and composite multilayers. Apart from structural properties, the stability, lubrication and biological functions of such layers were investigated. These data allow identification of new routes for designing drug delivery systems optimised for targeted oral deposition [150]. The composition and structure of salivary films depend on location in the oral cavity and the nature of the underlying oral substrates, that is, keratinised versus nonkeratinised tissues, hydrophobic versus hydrophilic areas. In general, the bare oral mucosa is a largely hydrophobic surface, which becomes more hydrophilic as proteins bind [151]. Inter- and intra-individual variation [152] can also alter pellicle development, where considerable differences in the protein profile and protein concentration effect changes in the pellicle composition [153]. Despite diversity, there are key features that are captured in a schematic diagram representing a 3D model of a salivary film formed on soft surfaces (Figure 1.4). Firstly, salivary proteins have the ability to bind directly to the mucosa epithelial layer. This process is often triggered by pre-cursor pellicle proteins. This is seen within the enamel pellicle, where proteins such as statherin and proline-rich proteins are thought to initiate pellicle formation [154,155]. On soft tissues, the evidence suggests that mucin–mucin interactions between salivary MUC5B [156] and membrane-bound MUC1 are a likely trigger mechanism of initial stages of mucosal pellicle formation [157]. There is evidence that covalent links mediated by transglutaminase, which catalyse cross-links between glutamine and lysine residues, may contribute to the attachment of this initial precursor film to the epithelium; however, this may not be a universal mechanism [99,154,155]. Figure 1.4 Schematic 3D model of a salivary film formed on soft surfaces (see text for details). Further build-up of the pellicle is facilitated by quickly adsorbing protein moieties. This process results in a multimolecular layer of tightly bound lower molecular weight proteins being formed adjacent to the epithelium. This layer comprises proline-rich proteins (primarily acidic), cystatins, histatins and statherin. The latter has a particular affinity to the hydroxyapatite of tooth enamel due to calcium binding domains. Other synergistic interactions, such as heterotypic complexes formed between sIgA and lactoferrin with MUC7, contribute to improved protein binding within the pellicle and the immunoprotective properties of the mucosal pellicle layer [77,158]. Along with lower molecular weight proteins, the nonglycosylated parts of MUC5B become trapped or anchored within this layer. With little room to manoeuvre, the slow diffusing glycosylated parts of mucins become protruded into the liquid salivary film, thus resulting in the formation of a highly hydrated and diffuse outer layer. The structure of this layer bears similarity with surface brushes, and also may contain loops of mucin chains with liquid water trapped within. Such architecture is key for lubrication, as it results in a highly hydrated and thick (up to 100 nm) layer with high load-bearing capacity, due to the increased viscoelastic response of the layer [159,160]. Thus, it is an effect of co-adsorption of low and high molecular weight proteins that results in a multilayer assembly that provides both strong adhesion to the oral surfaces and hydration and lubrication functionality. This structural model has been corroborated in a number of studies using both saliva as well as model systems [161,162]. AFM microscopic studies of saliva pellicle formation on aluminium surfaces also suggested a gradual build-up of the pellicle [163]. Initially, a thin (about 4 nm) layer with high fraction of proteins (∼40 v/v %) forms; this is followed by formation of a relatively thick (about 30 nm) and diffuse layer (∼1.5 v/v %). This diffuse layer stabilises after approximately 12 h of equilibration time. The fact that a thin layer forms faster is in agreement with the general adsorption behaviour of polymers, whereby the smaller and faster diffusing molecules have an advantage compared to larger and slowly diffusing molecules. The latter, however, may have higher surface affinity and, therefore, with time replaces initially adsorbed proteins [164]. The constant exchange of material with the bulk of the salivary films keeps the pellicle regenerated and maintains its integrity [165]. In addition to secretory components, salivary films contain a number of highly variable admixtures, such as residual food particles, as well as carbohydrates and proteins of bacterial origin. The pellicle physicochemical properties are sensitive to the environment and reflect several oral pathological states, such as erosion, periodontal disease and so on. [166]. Factors such as diet, circadian cycles, microbial flora, state of hydration, medication and psychophysiological conditions (e.g. stress) influence formation and properties of salivary pellicle [167]. This puts an additional strain on studies of salivary films, as it is important to control multiple parameters at once. The examination of ex vivo saliva samples is a common route to investigate pellicles and thin film salivary properties. Although it is realised that saliva changes its properties very shortly after expectoration, the ex vivo studies enable more controlled experiments with access to a more powerful suite of characterisation techniques. The mechanistic insights generated using ex vivo pellicle have to be treated with caution; however, most of the fundamental physicochemical insights can be translated to in vivo pellicles. Whole human saliva can be readily expectorated and collected. The use of collection devices is required for the collection of the gland specific salivary secretions. Salivary proteins are readily adsorbed on any type of surfaces, therefore binding should be minimised by either using low protein binding receptacles, for example PEGylated, or containers with the a surface-to-volume ratio. For many saliva diagnostic purposes cotton swabs are used, which is a convenient way that, however, may render inaccurate representation of protein composition due to adsorption of proteins on cellulose fibres. In most of cases, saliva should be collected on ice to reduce proteolytic and bacterial activity. The resting saliva can be collected from subjects that drool their saliva in a receptacle. To do that, the panellists usually should refrain from eating or consuming beverages (even water) for at least two hours prior to collection. The collection should happen at the same time of the day to minimise the effects of circadian variation. Participants are requested to sit comfortably with head tilted slightly forward so that saliva was able to pool into the front of the mouth. They should drool saliva without forcing it or agitating it with their cheeks or tongue to prevent any shearing of the sample. The collection process can take up to five minutes, with the collected volumes ranging anywhere between 1 and 3 ml. The collection of mechanically stimulated saliva can be conducted using a flavourless chewing gum base, paraffin wax or a piece of silicon tubing. Paraffin has been used for a long time but it has an unpleasant taste and contains contaminants. The use of chewing gum seems to be more natural, but even specially fabricated gum bases contain polyols, such as sorbitol, that may interfere with the collection. The use of silicon tubing has become more widespread, especially with the availability of high purity medical silicone material. During the collection process, the first portion of saliva should discarded due to the higher likelihood of adulterating components leaking from chewing gum or tubing. Then participants expectorate saliva in 30-second intervals into a pre-weighed container. Mechanically stimulated saliva contains a larger proportion of parotid secretions, as parotid glands are stimulated by chewing. The collection of acid stimulated saliva is typically done using a solution of citric acid (0.1–0.25 wt-%) or more concentrated citric acid drops (∼2 wt-%). When solution is used it is swirled around the mouth and the first two portions of expectorated sample are discarded before collecting saliva samples with minimal citric acid contamination. With more concentrated drops these are placed on the dorsal surface of the tongue and special care is taken to keep the acid solution away from the collection area. Acid stimulated saliva contains larger proportions of sublingual and, to some extent, submandibular secretions. Collection of saliva from minor glands can be challenging due to extremely low volumes and the poor accessibility of some glands (e.g. glossopalatine glands). Secretions from more accessible labial glands can be collected using swabs and microcapillaries. Collected saliva can be further processed, although any processing step affects the saliva film properties, especially its rheological properties [168], which are particularly vulnerable with regard to time-dependent degradation, with changes occurring just minutes after collection. The effect of ageing is particularly apparent on the microstructure of saliva, with aged saliva samples having more aggregated proteins in a form of salivary micelles. Saliva dilution also affects its microstructure, and hence the physical properties of salivary films. Centrifugation to remove debris can be used with accelerations <1000 g for 10–20 min, although care must be taken to avoid warming due to air friction. Filtering through a cotton wool, gauze or plastic mesh (like, e.g., cell strainer meshes) or fibre glass is also commonly used although some salivary proteins may bind to the cotton matrix. It is advisable to condition filter material in saliva prior filtering the sample intended for investigation. In general, the conditioning step should be used whenever practical. For example, tubing, measuring cells and chambers, or any other parts of instrument that may be exposed to saliva should be conditioned prior to using with samples. Finally, many reports feature addition of protease inhibitors that are added to the collected samples. This practicality of this step is key for analytical work on saliva and biomarker search. However, for physical characterisation the influence of a protease inhibitor cocktail remains debatable, since rheological properties deteriorate regardless of protease inhibitors, whilst friction properties remain largely unaffected. Ductal secretions from parotid salivary glands can be collected using a Lashley cup device [169]. Secretions from sublingual and submandibular ducts and be collected using moulded devices with the position of tubing apertures made to match duct openings. There are a number of different designs like Block and Brotman [170], Schneyer [171], and Truelove, Bixler and Merritt [172] collectors. There are somewhat simpler devices (e.g. Wolff et al. [173]) that use a principle akin to gas wash bottles. In this device the tip of the collection tube is positioned against the duct opening and suction is applied through the second orifice of the device using a suction pump; saliva is then collected in a receptacle such as centrifuge vial. With some subjects whose ducts are anatomically more separated, it is possible to separate sublingual and submandibular secretions by manipulating the device and blocking other ducts with filter paper or cotton wool [174,175]. Rheological properties of salivary bulk thin films, that is, films with thicknesses of a few microns and above, are characterised by a very high elastic part component of saliva’s viscoelastic behaviour [168]. In that sense saliva is a highly non-Newtonian fluid especially at extremely low relaxation frequencies. This elasticity manifests itself macroscopically during, for example, extensional deformation when very well-known saliva strings are forming. Conventional G’ oscillatory rheological measurements indicate that a model with multiple relaxation times is required to explain saliva rheological behaviour. The non-Newtonian behaviour of saliva, contrary to expectations, is more pronounced at low frequency oscillations. Very low frequencies are difficult to access experimentally in oscillatory shear measurements; hence, G’ values do not always bear complete information about the system. By contrast, a primary normal stress difference, N1, measured in a steady shear flow experiment can provide better description of saliva elasticity. The stress ratio N1/σ becomes a useful parameter for describing the non-Newtonian behaviour of saliva. The flow curve results can be successfully modelled using the well-known FENE-P model (Finitely Extensional Nonlinear Elastic with Peterlin closure), which combines contributions from the solvent (ηs) and polymer (ηp) viscosity:
Oral Mucosa: Physiological and Physicochemical Aspects
1.1 Anatomical and Histological Aspects of Oral Cavity Tissues
1.1.1 Tissue Architecture
1.1.2 Innervation
1.1.3 Receptors
1.2 Production and Composition of Saliva
1.2.1 Major Salivary Glands
1.2.2 Minor Salivary Glands
1.2.3 Saliva Composition
1.2.4 Mucins
1.2.5 Proline-rich Proteins
1.2.6 Statherins
1.2.7 Cystatins
1.2.8 Histatins
1.2.9 Salivary Amylase
1.2.10 Diversity of Salivary Film
Saliva/mucosal surface
Mucosal surface condition
Thickness of mucosal fluid (μm)
Protein concentration (mg/ml)
Unstimulated whole-mouth saliva
n/a
n/a
3.07 ± 0.27
Anterior hard palate
Wet
9.6 ± 3.0
22.0 ± 5.5
Buccal mucosa
Wet
39.5 ± 7.4
7.1 ± 0.6
Dry
17.1 ± 3.4
19.6 ± 7.4
Anterior tongue
Wet
54.0 ± 5.8
3.3 ± 0.7
Dry
12.3 ± 2.2
12.5 ± 2.6
Lower labial mucosa
Wet
20.8 ± 2.5
22.2 ± 4.3
Dry
6.0 ± 0.6
41.3 ± 13.5
Saliva/mucosal surface
Thickness of mucosal fluid (μm)
Protein concentration (mg/ml)
Thickness of mucosal fluid (μm)
Protein concentration (mg/ml)
UWMS
n/a
1.8 ± 0.3
n/a
3.8 ± 0.9
Anterior hard palate
10.6 ± 2.6
4.6 ± 1.9
5.6 ± 1.8
57.4 ± 14.3a
Buccal mucosa
38.7 ± 5.9
3.9 ± 0.6
17.8 ± 4.4a
34.5 ± 17.1
Anterior tongue
68.6 ± 4.5
2.7 ± 0.9
37.8 ± 9.6a
18.9 ± 5.8a
Lower labial mucosa
24.6 ± 3.5
9.4 ± 1.9
13.5 ± 2.1a
18.7 ± 5.5
a Statistically significantly different (P < 0.05)* from that of age-matched controls.
UWMS: unstimulated whole mouth saliva.
1.3 Surface Architecture, Mechanical, Rheological and Transport Properties of Salivary Pellicle
1.3.1 Ex Vivo Pellicle
1.3.2 Saliva Collection and Handling
1.3.3 Rheology
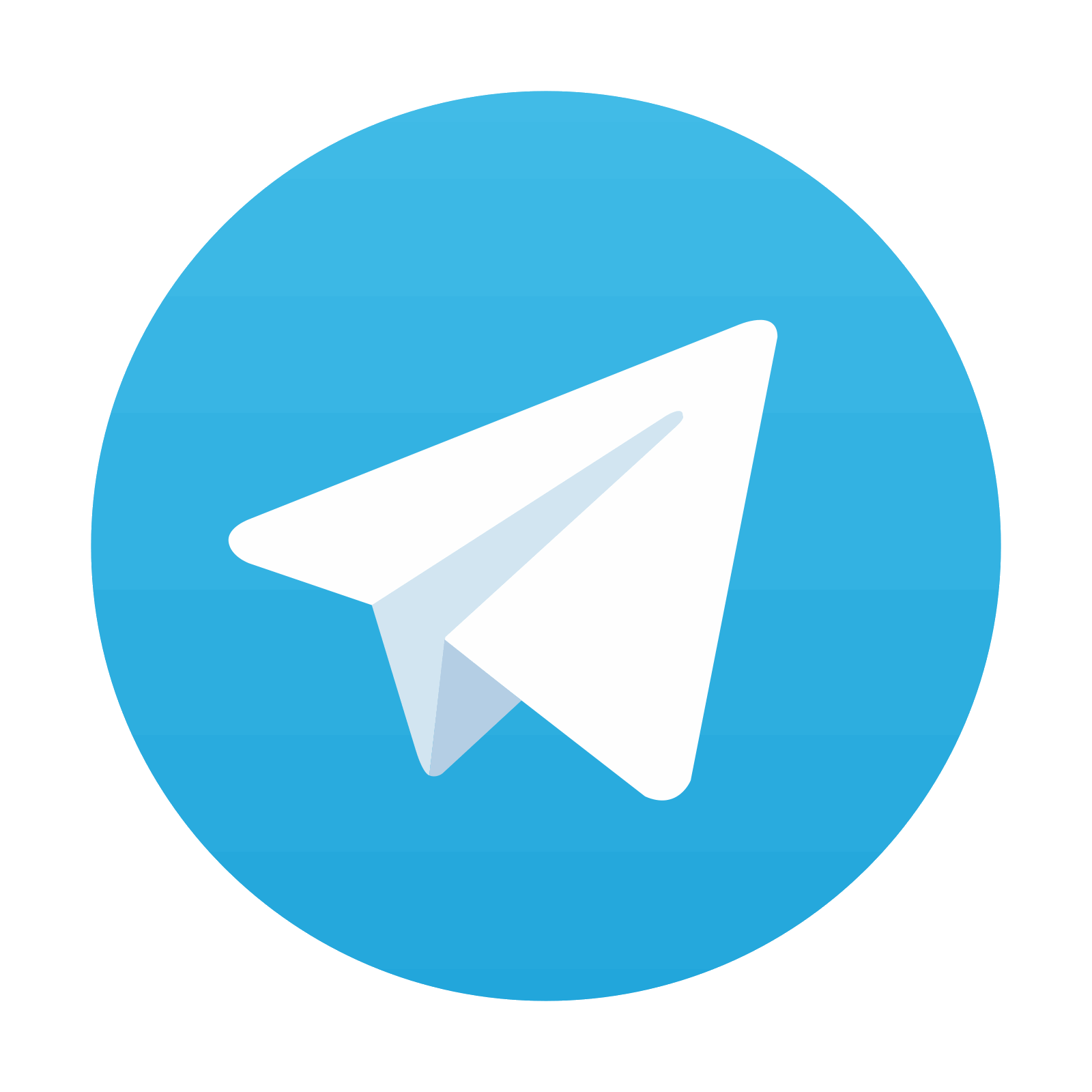
Stay updated, free articles. Join our Telegram channel
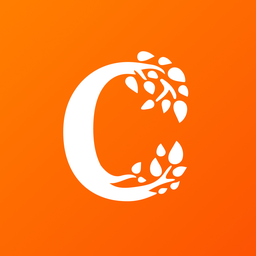
Full access? Get Clinical Tree
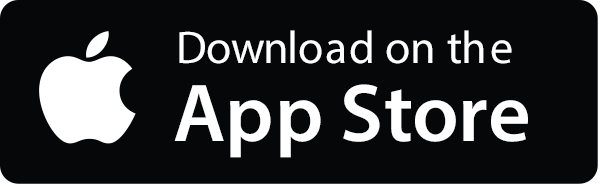
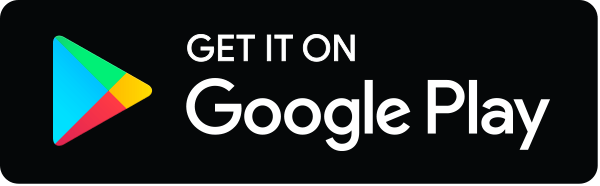