Chapter 10 Larry J. Kricka, D.Phil., F.A.C.B., C.Chem., F.R.S.C., F.R.C.Path. and Jason Y. Park, M.D., Ph.D., F.C.A.P.* where c = speed of light in a vacuum (3 × 1010 cm/s), and λ = wavelength in centimeters. Combining equations (1) and (2) results in The human eye is able to detect radiant energy with wavelengths between about 380 and 750 nm, but modern instrumentation permits measurements at both shorter wavelength (UV) and longer wavelength (IR) portions of the spectrum. Sunlight, or light emitted from a tungsten filament, is a mixture of wavelengths or a spectrum of radiant energy of different wavelengths that the eye recognizes as “white.” Table 10-1 shows approximate relationships between wavelengths and color characteristics for the UV, visible, and short IR portions of the spectrum. Thus a solution will appear green when viewed against white light if it transmits light maximally between 500 and 580 nm but absorbs light at other wavelengths. Similarly, a solid object appears green if it reflects light in this region (500 to 580 nm) but absorbs light at other portions of the spectrum. In general, if we compare the intensity of light transmitted by a colored solution versus that of a reference solution over the entire spectrum, we obtain a typical spectral transmittance curve characteristic for that spectrum. Such curves are shown in Figure 10-1 for solutions of nickel sulfate (a) and potassium permanganate (b). Inspection of the curves should lead us to predict that the color of solution a is green because light is transmitted maximally near the green portion of the spectrum. Curve b, on the other hand, illustrates the spectrum of a solution that transmits light maximally in the blue, violet, and red portions of the spectrum. The eye recognizes this mixture of colors as purple. TABLE 10-1 Ultraviolet, Visible, and Short Infrared Spectrum Characteristics *Owing to the subjective nature of color, the wavelength intervals shown are only approximations. †The ultraviolet (UV) portion of the spectrum is sometimes further divided into “near” UV (200-380 nm) and “far” UV (<220 nm). This arbitrary distinction has a practical basis, because silica used to make cuvets transmits light effectively at wavelengths ≥220 nm. A portion of the incident light, however, may be reflected by the surface of the cell or may be absorbed by the cell wall or solvent. To focus attention on the compound of interest, elimination of these factors is necessary. This is achieved using a reference cell identical to the sample cell, except that the compound of interest is omitted from the solvent in the reference cell. The transmittance (T) through this reference cell is IR divided by IO; the transmittance for the compound in solution then is defined as I divided by IR. In practice, the light beam is blocked and the detector signal set to zero transmittance, then a reference cell is inserted and the detector signal adjusted to an arbitrary scale reading of 100 (corresponding to 100% transmittance), followed by the cell containing the sample to be measured, and the percent transmittance reading is made on the sample. As we increase the concentration of the compound in solution, we find that transmittance varies inversely and logarithmically with concentration (Figure 10-2). Consequently, it is more convenient to define a new term, absorbance (A), which will be directly proportional to the concentration. Thus the amount of light absorbed (A) as the incident light passes through the sample is equivalent to Beer’s law (also known as the Beer-Lambert law) states that the concentration of a substance is directly proportional to the amount of light absorbed or inversely proportional to the logarithm of the transmitted light (see Figure 10-2). Mathematically, Beer’s law is expressed as a = Proportionality constant defined as absorptivity c = Concentration of the absorbing compound, usually expressed in moles per liter This equation forms the basis of quantitative analysis by absorption photometry. When b is 1 cm and c is expressed in moles per liter, the constant a is called the molar absorptivity. The value for a is a constant for a given compound at a given wavelength under prescribed conditions of solvent, temperature, pH, and so forth. The nomenclature of spectrophotometry is summarized in Table 10-2. Values for a are useful for characterizing compounds, establishing their purity, and comparing the sensitivity of measurements obtained on derivatives. Pure bilirubin, for example, when dissolved in chloroform at 25 °C, has a molar absorptivity of 60,700 ± 1600 at 453 nm. The molecular weight of bilirubin is 584. Hence a solution containing 5 mg/L (0.005 g/L) should have an absorbance of TABLE 10-2 Spectrophotometry Nomenclature *I/I0 is the ratio of the intensity of transmitted light to incident light. In some cases, a pure reference material may not be readily available, and constants may be provided that were obtained on pure materials and reported in the literature. In general, published constants should be used only if the method is followed in detail and readings are made on a spectrophotometer capable of providing light of high spectral purity at a verified wavelength. Use of broader-band light sources usually leads to some decrease in absorbance. For example, the absorbance of nicotinamide adenine dinucleotide (NADH) at 340 nm is frequently used as a reference for determination of enzyme activity, based on a molar absorptivity of 6.22 × 103 (see Chapter 15). This value is acceptable only under the carefully controlled conditions previously described and should not be used unless these conditions are met. Published values for molar absorptivities and absorption coefficients should be used only as guidelines until they are verified by readings on pure reference materials for a given instrument. In addition, Beer’s law is followed only if the following conditions are met: • Incident radiation on the substance of interest is monochromatic. • The solvent absorption is insignificant compared with the solute absorbance. • The solute concentration is within given limits. • An optical interferant is not present. • A chemical reaction does not occur between the molecule of interest and another solute or solvent molecule. The major components of a single-beam spectrophotometer are shown schematically in Figure 10-3. In such an instrument, a beam of light is passed through a monochromator that isolates the desired region of the spectrum to be used for measurements. The light next passes through an absorption cell (cuvet), where a portion of the radiant energy is absorbed, depending on the nature and concentration of the substance in the solution. Any light not absorbed is transmitted to a detector, which converts light energy to electrical energy that is registered on a meter or recorder or digitally displayed. Figure 10-4 illustrates schematically a typical double-beam instrument that uses a light-beam chopper (a rotating wheel with alternate silvered sections and cutout sections) inserted after the exit slit. A system of mirrors passes portions of the light reflected off the chopper alternately through the sample and a reference cuvet onto a common detector. The chopped-beam approach, using one detector, compensates for light source variation and for sensitivity changes in the detector. Incandescent, Arc, and Cathode Lamps: The light source for measurements in the visible portion of the spectrum is usually a tungsten light bulb. The lifetime of a tungsten filament is greatly increased by the presence of a low pressure of iodine or bromine vapor within the lamp. An example is the quartz-halogen lamp, which has a fused-silica envelope and which provides high-intensity light over a wide spectrum for extended operating periods (2000 to 5000 hours) before replacement is necessary. Mercury arc lamps emit an intense 254-nm resonance line and are widely used as detectors in high-performance liquid chromatography (HPLC) (see Chapter 13). Alternatively, some HPLCs employ a miniature hollow cathode lamp as a very-narrow-wavelength intense source. For example, a zinc hollow cathode lamp emits a line at 214 nm that is close to the maximum wavelength of peptide bond absorption (206 nm); this emission property permits the usage of such lamps to measure peptides and proteins. Details on the hollow cathode lamp are found in the section on “Atomic Absorption Spectrophotometry.” The hollow cathode lamp also has a long, useful lifetime if a lower-current, nonpulsed power supply is used. Laser Sources: A laser (light amplification by stimulated emission of radiation) is a device that controls the way that energized atoms release photons; lasers are used as light sources in spectrophotometers because they provide intense light of a narrow wavelength. Through selection of different materials, different wavelength(s) of light are emitted by different types of lasers (Table 10-3). TABLE 10-3 Various Types of Lasers and the Wavelengths at Which They Operate Infrared diode lasers are used in compact disc players and laser printers, and in bar code readers (see Chapter 19). They are solid-state devices, typically constructed of gallium arsenide, and energy is pumped into them at a low potential of −1.5 V. Depending on its construction, the wavelength output of the laser ranges from 550 to 1810 nm. Development of inexpensive near-IR lasers has led to interest in using reflective techniques in the near-IR region of the spectrum (0.8 to 2.5 µm wavelength). Reflectance spectrophotometry is now used clinically for the transcutaneous measurement of bilirubin in neonates.14 Another application of reflectance spectrophotometry is measurement of blood oxygen saturation in near-IR and IR regions. Filters: The simplest type of filter is a thin layer of colored glass. Certain metal complexes or salts, dissolved or suspended in glass, produce colors corresponding to the predominant wavelengths transmitted. The spectral purity of a filter or other monochromator is usually described in terms of its spectral bandwidth. This is defined as the width, in nanometers, of the spectral transmittance curve at a point equal to one half the peak transmittance. Because glass filters have spectral bandwidths of approximately 50 nm, they are referred to as wide-bandpass filters. Prisms and Gratings: Prisms and diffraction gratings are widely used as monochromators. A prism separates white light into a continuous spectrum because shorter wavelengths are bent, or refracted, more than longer wavelengths as they pass through the prism. A diffraction grating is prepared by depositing a thin layer of aluminum-copper alloy on the surface of a flat glass plate, then ruling many small parallel grooves into the metal coating. Better gratings contain 1000 to 2000 lines/mm and must be made with great care. These are then used as molds to prepare less expensive replicas for general use in instruments. Selection of a Wavelength Isolation Device: The type of monochromator chosen depends on the analytical purpose for which it is to be used. For example, narrow spectral bandwidths are required in spectrophotometers for resolving and identifying sharp absorption peaks that are closely adjacent. Lack of agreement with Beer’s law will occur when a part of the spectral energy transmitted by the monochromator is not absorbed by the substance being measured. This is more commonly observed with wide-bandpass instruments. In practice, an increase in absorbance and improved linearity with concentration are usually observed with instruments that operate at narrower bandwidths of light. This is especially true for substances that exhibit a sharp peak of absorption. Photomultiplier Tubes: A photomultiplier tube (PMT) contains (1) a cathode, (2) a light-sensitive metal, and (3) a series of dynodes, all of which are enclosed in an evacuated glass enclosure. As many as 10 to 15 stages or dynodes are present in common photomultipliers. Photons that strike the photoemissive cathode emit electrons that are accelerated toward the dynodes. Additional electrons are generated at each dynode. Depending on the number of dynodes and the accelerating voltage, the cascading effect creates 105 to 107 electrons for each photon hitting the first cathode. This amplified signal is finally collected at the anode, where it can be measured. When such a tube is operated, voltage is applied between the photocathode and each successive stage. The normal incremental increase in voltage at each photomultiplier stage is from 50 to 100 V larger than that of the previous stage (Figure 10-5). Typically, a conventional PMT tube has approximately 1500 V applied to it. Photodiodes: Photodiodes are solid-state photodetectors that are fabricated from photosensitive semiconductor materials such as (1) silicon, (2) gallium arsenide, (3) indium antimonide, (4) indium arsenide, (5) lead selenide, and (6) lead sulfide. These materials absorb light over a characteristic wavelength range (e.g., 250 nm to 1100 nm for silicon). Their development and use as detectors in spectrophotometers have resulted in instruments capable of measuring light at a multitude of wavelengths. When a photodetector consists of two-dimensional arrays of diodes, it is known as a photodiode array. Each photodetector within the array responds to a specific wavelength. For example, photodiode arrays have been designed to have a 2-nm resolution per diode from 200 to 340 nm, and a 1-nm resolution per diode from 340 to 800 nm. Electrical energy from a detector is displayed on some type of meter or readout system. In the past, analog devices were widely used as readout devices in spectrophotometers. However, they have been replaced by digital readout devices that provide a visual numeric display of absorbance or converted values of concentrations. Spectrophotometers may be equipped with recorders in addition to or instead of a digital display. These are synchronized to provide line traces of transmittance or absorbance as a function of time or wavelength. When a continuous tracing of absorbance versus wavelength is recorded, the resultant display is called an absorption spectrum. If a substance absorbs light, distinct peaks of absorbance will be observed (see Figure 10-1). Measuring the absorption spectra of an unknown sample and comparing them with spectra from known compounds is very useful for qualitative purposes. For example, this type of procedure is especially useful for identification of drugs that absorb in the UV region. The National Institute of Standards and Technology (NIST) provides several standard reference materials (SRMs) for spectrophotometry that are useful in the calibration or verification of the performance of photometers or spectrophotometers (e.g., SRM 930e is for the verification and calibration of the transmittance and absorbance scales of visible absorption spectrometers) (http://www.nist.gov, accessed March 23, 2011). The Institute for Reference Materials and Measurements (IRMM) belongs to the European Commission and provides reference materials for verification of the performance of photometers or spectrophotometers. These materials are listed in the IRMM ERM Reference Materials Catalogue (http://www.irmm.jrc.be, accessed March 23, 2011). With narrow-spectral-bandwidth instruments, a holmium oxide glass may be scanned over the range of 280 to 650 nm. This material shows very sharp absorbance peaks at defined wavelengths, and the operator may compare the wavelength scale readings that produce maximum absorbance with established values. If compared values do not coincide, a calibration correction table can be constructed to relate scale readings to true wavelengths. A typical spectral transmittance curve for holmium oxide glass is shown in Figure 10-6. Selected absorption peaks for this filter, which are suitable for calibration purposes, occur at the following wavelengths: 279.3, 418.5, 287.6, 536.4, 333.8, 637.5, and 360.8 nm. Solutions of holmium oxide in dilute perchloric acid have also been recommended and may be used with any spectrophotometer. With broader-bandpass instruments, a didymium filter may be used to verify wavelength settings. This filter should show a minimum percent transmittance at 530 nm against an air blank (Figure 10-7). Because didymium has several absorption peaks, the setting should be verified grossly by visual examination of transmitted light. This light should appear green at 530 nm.
Optical Techniques
Nature of Light
Wavelength, nm
Region Name
Color Observed*
<380
Ultraviolet†
Invisible
380-440
Visible
Violet
440-500
Visible
Blue
500-580
Visible
Green
580-600
Visible
Yellow
600-620
Visible
Orange
620-750
Visible
Red
800-2500
Near infrared
Not visible
2500-15,000
Mid infrared
Not visible
15,000-1,000,000
Far infrared
Not visible
Spectrophotometry
Basic Concepts
Beer’s Law—Relationship Between Transmittance, Absorbance, and Concentration
Name
Symbol
Definition
Absorbance
A
logT or logI0/I
Absorptivity
a
A/bc (c in g/L)
Molar absorptivity
ε
A/bc (c in mol/L)
Path length
b
Internal cell or sample length, in cm
Transmittance
T
I/I0*
Wavelength unit
nm
10−9 m
Absorption maximum
λmax
Wavelength at which maximum absorption occurs
Application of Beer’s Law
Instrumentation
Components
Light Sources
Laser
Wavelengths, nm
Argon fluoride
193
Argon fluoride
248
Helium-cadmium
325 or 442
Nitrogen
337
Argon (blue)
488
Argon (green)
514
Helium-neon (green)
543
Light-emitting diode—GaP
550 or 700
Rhodamine 6G dye (tunable)
570-650
Laser diode (AlGaInP, GaAlAs)
633-1660
Helium-neon (red)
633
Ruby (CrAlO3) (red)
694
Light-emitting diode—GaAs
880
Light-emitting diode—Si
1100
Neodymium-YAG (yttrium aluminum garnet)
1064
Carbon dioxide
9300, 9600, 10,300, or 10,600
Spectral Isolation
Photodetectors
Readout Devices
Performance Parameters
Wavelength Calibration
Stay updated, free articles. Join our Telegram channel
Full access? Get Clinical Tree
Optical Techniques
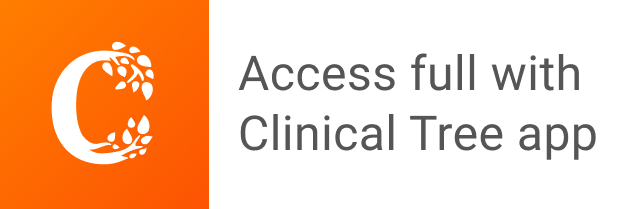