9 Gleb E. Yakubov1,2,3, Scott Singleton3 and Ann-Marie Williamson3 1School of Chemical Engineering The University of Queensland Australia 2Australian Research Council Centre of Excellence in Plant Cell Walls, The University of Queensland, Australia 3Unilever R&D Colworth UK Mucins are ubiquitous glycoproteins that can be found in all metazoan species [1]. They form a glycocalyx layer around all animal cells and are a key component of mucus in all mucosal tissues [1a]. The general principle of mucin structure comprises a protein backbone decorated with a bottle brush of oligosaccharide side chains [2]. Membrane-bound mucins that form glycocalyx are di-blocks; they have a membrane-bound nonglycosylated domain and a glycosylated domain facing out into intercellular space [1a]. Secreted mucins are typically tri-blocks, whereby one or more heavily glycosylated domains are confined between terminal domains that are largely nonglycosylated [1a,3]. Secretory mucins are a major part of various mucosa linings: gastric, intestinal, respiratory, oral, ocular, urogenital and so on [1a]. The key property of mucins is the ability to form a network via end-to-end association promoted by disulfide bonds, entanglement and colloidal interactions such as electrostatic and hydrophobic [2,4]. When adsorbed they can form multilayers and adsorb onto a wide range of surface chemistries [5]. The architecture of mucosa depends on the type and combination of mucins with other components. For example, in saliva mucins complex with other salivary proteins, notably proline-rich proteins; such supramolecular assemblies form salivary pellicle that coats all surfaces in the mouth including teeth and the tongue [6]. The common structural motif of mucosa is a porous structure formed by a mucin network. Most of mucosal tissues are also characterised by a high level of hydration (90–98%) [7]. From a polymer physics perspective, mucins are hydrophobic block copolyampholytes with net negative charge. Mucin’s ‘naked’ protein backbone comprises hydrophobic and positively as well as negatively charged domains. Many carbohydrate side chains attached to a proline-rich part of the backbone are negatively charged due to presence of terminal sialic acid or sulfate-modified carbohydrate residues [8]. The structural integrity of such chemically heterogeneous molecules is secured by highly hydrated glycosylated regions that give mucins sufficient rigidity without compromising the mechanical flexibility of the network [9]. Most mucosal tissues are exposed to aggressive environments, including the majority of the external surfaces not covered by skin. Under excessive stress mucosa stability can be easily compromised. For example, many food polyphenols can bind to salivary components and perturb lubrication of salivary pellicle resulting in astringent mouth feel [6c,10]. To overcome the consequences of constant stress, mucosa is perpetually regenerated and, hence, is one of the most dynamic tissues. One of the key challenges in mucoadhesion is to overcome the perpetual flow of mucosa washing away any foreign residues. Measurement techniques employed for mucoadhesive studies should, consequently, possess the same progressive character to capture dynamic interactions. Complexity of mucin molecules permits multiple interaction mechanisms ranging from covalent chemical bonds to physicochemical and colloidal interactions. In real settings, the majority of mucoadhesive materials and delivery systems act concomitantly through multiple mechanisms, resulting in complex compounding interactions with mucosa. Key mechanisms targeted in mucoadhesive technologies are outlined briefly here. Electrostatic interactions act primarily between negatively charged sialic/sulfuric acid residues localised at termini of the oligosaccharide side chains. The pKa of sialic acid is 2.0–2.6 (depending on neighbouring oligosaccharide linkage) [9,11], hence under the majority of conditions (except gastric) mucins will bind to positively charged molecules. This interaction can be very strong due to high density of sialic acid residues. The ubiquitous use of chitosan, a positively charged polysaccharide, in mucoadhesive materials relies heavily on this mechanism [12]. Negatively charged molecules can also bind to mucins via positively charged amino acids present in the terminal (nonglycosylated) domains. The use of acrylates that like mucins otherwise are negatively charged partially relies on this mechanism [13]. The ionic environment has a strong impact on electrostatic interactions. For two charged ions or small molecules the electrostatic double layer force decreases with increasing electrolyte concentration (i.e. ionic strength) approximately following an exponential decay function [14]: where Many mucosa tissues are hypotonic relative to blood with pH ranging between 5 and 7. However, along the gastrointestinal tract the ion environment and pH do change significantly. For mucoadhesive systems targeting digestive release, it is therefore important to account for such variations and the use of polyampholytes and block copolymers is a promising route to overcome possible shadowing of electrostatic interactions in the different parts of the GI tract [15]. Hydrogen bonding is ubiquitous and can involve both carbohydrate and naked protein domains [12,16]. Although the energy of hydrogen bonds is lower compared to covalent bonds, hydrogen bonding can be copious, leading to high total binding energies. The complication with hydrogen bonding is to ensure synergistic binding and formation of many bonds at the same time. The certain repeat pattern and intramolecular spacing of functional groups is required for molecules to super-coil [17]. Even more stringent topological arrangements are required to allow helix-type structures. Mucins have previously been viewed as nonstructured or weakly structured polymers; hence, collective hydrogen bonding was not immediately anticipated. However, a number of reports suggested PPII-type structuring for nonglycosylated proline-rich parts of mucin protein backbone, which provides evidence that topology of functional groups indeed permits structuring through a cooperative arrangement of hydrogen bonds [18]. Hydrophobic interactions are evident from adsorption studies of mucins on hydrophobic surfaces [19] and from studies of mucin adsorption on oil droplets [20]. It has also been demonstrated that the hydrophobic interaction is one of the key mechanisms participating in the tail-to-tail aggregation of mucins. Hydrophobic amino acids can be found throughout the protein backbone and, especially, within ‘cysteine knots’, C- and D-domains located at both termini [1a]. The effectiveness of hydrophobic interaction is due to high energy and low sensitivity to the surrounding conditions. In the gastric environment where the pH is low, the hydrophobic interaction may take on the leading role amongst the physical interactions due to electrostatics being significantly supressed. Entanglement of polymeric materials provides an additional physical mechanism operational in mucosal deposition [21]. Most polymers can easily interpenetrate mucus gel and become entangled with mucin molecules. Polymer entanglement can provide robust adhesiveness with advantages coming from the dynamic nature of the molecular links. Yet entanglement can be attained only at relatively high mucin concentrations, that is significantly above overlap concentration ( Lectins are known to selectively bind carbohydrate residues and whole oligosaccharide domains. The interaction can be used especially effectively for membrane-bound mucins for targeted delivery to the cells at specific tissue localisations [22]. However, toxicity and immunogenic aspects of natural lectins limit their wide applicability. A promising route of using lectin epitopes that recognises their respective carbohydrate ligand has been suggested [23] but not fully demonstrated in practice. Chemical covalent interactions in mucins are chiefly due to the presence of cysteine rich domains (e.g. cysteine knots) that provide convenient anchors for thiols with forming disulfide covalent bonds. Thiol-based polymers (including thiolated chitosan) have been widely evaluated as advanced mucoadhesive agents [24]. The formation of disulfide bonds is capable of increasing the binding energy up to 1000 times [24a]. However, if binding is too strong it can compromise mucosa structure and, consequently, its barrier and lubricating capacity [10c]. Despite the high strength of chemical bonds the residence time attainable is still limited by mucosa dynamic turnover. The 3D assemble of mucosa has one critical function, that of providing barrier and protection of underlying tissues without impeding water transport. The thickness of mucus layer depends on location and varies from a few nanometres of mucin monolayers (e.g. on the sclera) to submillimetre thick mucosa linings in the thick intestine and colon [25]. The characteristic pore size distribution can also vary, typically of the order of 50–500 nm. Such submicron porous structure enables mucus to function as a size exclusion filter, preventing penetration of particulate materials and bacteria but allowing passage of smaller nutrient molecules and water [26]. The amphiphilic nature of mucins adds another dimension to this barrier functionality by enabling entrapment of moieties of either charge, even with sizes significantly smaller than that of the pores [27]. It has been shown that strongly charged particles of either charge have up to an order of magnitude lower diffusivity in the acidic mucus gels compared to their less charged analogues [27]. However, the mucus system has a ‘loop hole’, as it appears indifferent towards amphiphiles, that is molecules and particles that like mucins have positively and negatively charged domains. Some viruses use this ‘loop hole’ and have evolved to produce capsid materials with amphiphilic properties. This enables them to trick the systems and range through the mucosa barrier with little resistance [28]. Adhesion of particulates results in deformation of mucosa surfaces. For soft solids and semi-solid materials, the adhesion process is coupled with spreading of adhered material over mucosa substrates. Spreading expands the effective contact area and, hence, facilitates retaining of the material due to an increased number of molecular links per unit of adhered material [29]. Formation of adhesive contact and rheological behaviour of such links plays an important role in assessing the work of adhesion as well as in mediating the tribological properties of the mucus layer [30]. For soft adhesive contacts the total work of adhesion is a sum of the work required to break intermolecular bonds and the work of deformation of the mucosa substrate and the adhered material itself. hence, pull-off force is a superposition of contact mechanics and filament stretching The exact treatment of the problem depends on the type of strain function applied and measurement apparatus used. Thus for cantilever-based methods (e.g. AFM or SFA) the solution becomes quite complex, since the initial stretching rate of a filament depends on acceleration of the cantilever at the point of detachment; hence, the adhesive part and stretching part become kinematically coupled. The well-known lubricating effect of mucins and mucin-like glycoproteins provides active protection against wear in most moving parts such as joints, eye lids and in the mouth [31]. One of the key elements contributing to the robustness of mucosal barrier is its repair mechanism. The fresh mucosa is constantly synthesised and replaces the worn out material [7b,32]. This process puts fundamental time constraints on how long mucoadhesive material can reside at mucosa before washing out. The mucosal supramolecular structure is in part kinetically stabilised. Mucoadhesive interactions can easily shift the balance and mucus functionality can be incapacitated. Interaction may lead to dehydration and collapse of mucus gel. Hence, very strong attachment of the material is not always desirable. Attachment has to be just enough for materials to linger for a desired period of time. Therefore, use of multiple mechanisms and optimisation of interaction strength that is ‘just right’ is the key to achieve successful mucosal deposition. Multiple interaction mechanisms as well as a wide span of interaction length and timescales require not one but a suite of methods to investigate mucoadhesive interactions. An emphasis is put on novel developments in both in vitro and in vivo methods as well as development of functional assays for screening candidate molecules and delivery vehicles. In this section, major classes of methods that have been used for studying mucoadhesive interactions are outlined; short overviews of some molecular techniques are summarised in Table 9.1. Table 9.1 Summary of physical techniques used in probing mucoadhesive interactions at the molecular level. Ellipsometry, Spectroscopic Ellipsometry [33b,62a] ·chitosan [21b] ·polyacrylate (PAA) [85] ·lactoferrin/chitosan [86] ·cellulose derivatives [34a] ·poly(ethylene glycol) (PEG) [34b,87] ·chitosan [34b,87a] ·carbopol [37] ·polysaccharides [48b] ·lectin [88] ·pectin [87c] ·hyaluronic acid (HA), ·carboxymethyl cellulose (CMC), ·hydroxypropylmethyl cellulose (HPMC), ·polyamidoamine (PAMAM) [37,87c] ·chitosan [37] ·dextran, ·hydroxypropylcellulose (HPC) [89] ·lactoperoxidase [87c] · HA · CMC · HPMC · PAMAM [21b,90] ·PAA [33a,46a,c,62a,91] ·chitosan [23] ·lectins [46c] ·carbopol, ·HPMC [46d,88] ·pectin [85] ·lactoferrin [92] ·beta-cyclodextrin grafted with chitosan [93] ·PAA [94] ·poly(2-(dimethylamino-ethyl) methacrylate [34b,46b,95] ·chitosan [34b] ·carbopol [96] ·single molecule imaging [97] ·pectin [98] ·PAA-PMMA [99] ·chitosan liposomes [100] ·poly (3-acrylamidophenylboronic acid), ·poly (2-lactobionamidoethyl methacrylate) [33b,41,101] ·chitosan [13b] ·PAA ·Pluronic [102] ·PEG-(DOPA) [103] ·lactoperoxidase [42a] ·N-isopropyl-acryamide/glycidyl-acrylamide copolymer [33b] ·chitosan [19,42b,104] ·mucin interactions FTIR, ATR-FTIR, Raman Spectroscopy, Raman Optical Activity [38,40] ·PAA [29] ·pectin [105] ·poly(N-hydroxyethyl)-DL-aspartamide, ·polyaspartylhydrazide [40] ·PAA [106] ·chitosan derivatives [107] ·alginate derivatives [78] ·chitosan/bile salts [108] ·chitosan derivatives [109] ·chitosan ·PAA Molecular interactions in mucoadhesive systems are commonly probed using surface characterisation techniques based on optical methods such as ellipsometry [33], surface plasmon resonance spectroscopy (commonly using a commercial Biacore® set-up) [34], Dual Polarisation Interferometry [35], Optical Waveguide Lightmode Spectroscopy [36] and a number of other techniques. The methods rely on the principle that the adsorbed material changes the optical properties of the interface, thus resulting in changes in light propagation that, in turn, can be used to monitor deposition of a material on the surface. In a typical experiment, mucosal material is first adsorbed on the surface; then mucoadhesive material is perfused though (the opposite sequence is also possible). Changes in the refractive index or polarisation resulting from the deposition of the material can be quantified. The strength of the mucoadhesive interaction is then inferred based on the amount of the material deposited on a model mucosa substrate and retained after surfaces are perfused with a buffer. In addition to optical methods, Quartz Crystal Microbalance techniques (including QCM with dissipation) enable monitoring hydration of an adsorbed layer, thus providing additional structural information [37]. A combination of optical and microbalance techniques was proven to be a powerful tool to investigate molecular interactions, as it enables independent quantification of the adsorbed amount and layer hydration. Binding measurements provide superior time resolution and can be effectively combined with spectroscopic techniques to gain more detailed information about the interacting chemistries [21b,37]. Vibrational spectroscopic techniques such as FTIR/ATR-FTIR [38] and Raman Spectroscopy [39] as well as Molecular forces can be measured directly using surface force measurement techniques such as Atomic Force Microscope (AFM) [41] or Surface Force Apparatus (SFA) (or Surface Force Balance, SFB) [19,42]. In a typical experiment, one of the surfaces is modified with a molecular layer of mucin (or other mucosal target), while the other is modified with a mucoadhesive material. In SFA (SFB) both substrates have the same geometry, while in AFM a flat surface is typically set to interact with a sharp tip or microscopic colloidal probe. In some studies the measurements of interaction between two identical microscopic particles were accomplished. The use of a particle–particle arrangement can offer some advantages, particularly minimising the substrate disparity problem, but at the expense of more laborious experimental procedure with significantly larger measurement errors. The result of the experiment is a force versus distance curve recorded during approach and retraction of surfaces. The approach part of the curve is governed by the interaction potential between surfaces, while on retraction the pull-off event can provide a basis for calculating adhesion energy in conjunction with a suitable contact mechanics model. The most frequently used models are the Johnson–Kendall–Roberts (JKR) [43], Derjaguin–Muller–Toporov (DMT) [44] and Maugis–Dugdale [45] models. The latter is computationally sophisticated and bridges JKR and DMT models as limiting scenarios. The contact part of the force–distance curve can also be analysed and use of an appropriate contact mechanics model can enable determination of the mechanical parameters of the materials, such as Young’s modulus, Poisson ratio and so on. In addition to direct force measurements, the AFM and Confocal Scanning Laser Microscope (CSLM) can be used to image mucosal materials [46]. Imaging data can also provide measures of mucoadhesive interactions, though in an indirect way. Observation and quantitative analysis of aggregates, their sizes and structures can be used to infer the strength and mechanism of mucoadhesive interactions. Unlike direct force measurements, imaging can provide a quicker and more versatile alternative, with much less stringent requirements for sample preparation. Recent progress has made it possible to study mucoadhesive interactions using isothermal titration calorimetry (ITC) [47]. The ITC method uses the thermal compensation principle (akin to the one used in differential scanning calorimetry) and enables characterisation of thermal effects associated with binding. In a typical experiment a solution of a polymer or protein is loaded in a thermostatically controlled chamber (typically 0.2–5 ml). The ligand solution is then loaded in a microsyringe equipped with a flat needle that is inserted into the chamber with the polymer solution. The syringe is connected to a pumping system that injects aliquots of ligand solution into polymer solution. Each injection results in a binding reaction that either produces or takes up the heat. A compensation mechanism keeps the temperature of the chamber constant relative to the reference. The amount of energy supplied to a thermostat jacket is then proportional to the heat released or consumed by the reaction. A series of sequential injections produce a binding curve, the dependency of the enthalpy on ligand/polymer molar ratio. The analysis of the experimental binding curves can be quite challenging, since the exact form of the binding equation is not known a priori. However, if successful, the method enables extraction of very valuable thermodynamic parameters of binding in the mucoadhesive system, such as binding enthalpy, binding constant and stoichiometry of binding. The technique has been successfully applied to study lectin binding [48] and formation of disulfide bonds for testing the efficiency of thiol-modified mucoadhesive polymers [49]. There are a number of requirements and limitations that should be considered when performing an ITC experiment. For example, solutions should have relatively low viscosity to minimise viscous drag effects and ensure quick equilibration. If interactions are weak, the analysis may not yield full thermodynamic characterisation [50]. Various rheological tests have long been used to assess mucoadhesive interactions and have been discussed in detail elsewhere [51]. For many mucosal membranes, such as oral and ocular, one has to consider two surfaces, for example the tongue and the pallet, the sclera and the eye lid that in normal physiology do come in contact and may implicate application of, for example, a mucoadhesive compound. Mucoadhesive interactions may impair lubrication and lead to adverse effects. For example, oral applications of chitosan is believed to be associated with binding to salivary proteins [52]. The investigation of lubrication can, therefore, provide a direct measure of potential impact of mucoadhesive ingredients on mucosal structure. In soft mucosal systems, effective measurements of lubrication can be conducted using soft-contact tribology techniques, where rubbing contact pressures do not exceed a few MPa, and hence do not lead to significant wear. Unlike conventional tribological methods, the use of soft elastomer surfaces or indeed excised tissues (including tongue and oesophagus) makes this methodology suitable for probing mucosal interactions [30,53]. Low contact pressure, large surface area, ability to control roughness, are key attributes of soft-contact tribology that enable effective mimicking of real mucosal contacts and biolubrication conditions. In a typical in vitro experiment, two elastomer surfaces are set in a rubbing contact in a bath filled with material or buffer. By introducing a mucoadhesive ingredient into the system it is possible to monitor the changes in friction force with time. There is also a possibility to controllably apply force, entrainment speed and type of motion between surfaces, ranging from pure sliding to almost pure rolling friction conditions. Macroscopic adhesion tests such as those using a Texture Analyser (or various custom-build apparatuses) tests have been routinely used to measure adhesive forces. The advantage of this type of method is the high throughput capacity, reliability and ability to accommodate various samples (including ex vivo tissues) [51]. The limitation of the methods stems from the fact that many interactions, such as, for example, capillary forces or wear effects, can be concomitant. The artefacts associated with such complex interactions are sometimes difficult to control, making accurate measurements of physical parameters extremely challenging. Macroscopic techniques, nonetheless, find a significant appeal if comparative investigations are in mind. In a typical experiment, one of the surfaces is a mucosal tissue or a modified substrate (typically metal or plastic). The probe can either be fabricated from a suitable material or likewise the substrate made of an excised tissue or mucosal sample. The result of the experiment is a force versus distance curve recorded during approach and retraction of surfaces. Typically, two regions of the curve are analysed; the indentation part enables measurement of elastic parameters of the substrates, and a pull-off region can be processed to yield adhesion energy data (the data processing is practically identical to that described for AFM/SFA force measurements). Another set of methods enabling macroscopic mucoadhesive measurements is retention methods, whereby a flow of the material (e.g. mucoadhesive candidate molecule) is forced to pass through a membrane modified with mucus materials or indeed through an excised mucosal tissue itself [54]. The transport measurements become of particular interest for comparative and benchmarking studies, and can be tuned to practically any type of mucosal substrates. In vivo methodologies provide a route for conducting a controlled mucoadhesive experiment in a setting closest to the real application. Recently an endoscopy technique, called in vivo-on mouth imaging, has been developed that can be used for measuring oral mucoadhesive interactions [55]. The detailed description of the technique has been reported elsewhere [56]. Briefly, the video-rate endoscopy technique [57] was combined with a positioning arm that accommodates all the major axes of movement of the subject. The system enables imaging of oral residues as a time-course measurement. The deposition data and material clearance rate can be readily extracted. Additionally, the device is equipped with a position control system that enables in situ control of anatomical position, enabling spatial data to be collected as well. The method in principle can be used for both fluorescent and white light illumination configurations. However, fluorescent images provide much better signal-to-noise ratio and are preferred. The use of fluorescent material may, in some cases, present a significant challenge but this method may play a key role in for accessing in vivo mucoadhesive data for the oral deposition applications. Magnetic Resonance Imaging (MRI) has been used to study retention of materials in the gastrointestinal tract to provide an access to the full scale anatomical and physiological features of the body [58]. The constantly increasing resolution of the MRI equipment [59] opens up a new spectrum of opportunities, whereby monitoring of localisation and passage of mucoadhesive delivery vehicles can be done at any location in the body. Examination in gastrointestinal tract, airways, cervix, urogenital tract, and indeed within tissues at the location of a potential delivery target may soon become possible in physiological studies of mucoadhesive interactions. For a number of years, mucoadhesive interactions have been the subject of investigation within the laboratory at Unilever R&D, with the main focus being to improve the taste and texture of foods. The leading hypothesis with respect to mucoadhesion was underpinned by a concept of rheological and tribological transformations of food materials during oral processing [60]. By combining the dynamic oral processing concept with engineered biosubstrate–product interactions, a way to achieve a sensorial benefit by exploiting a range of functional microstructures was sought.
Methods for Assessing Mucoadhesion: The Experience of an Integrative Approach
9.1 Mucins and Mucosal Architecture
9.2 Concept of Length and Time Scales in Mucoadhesion
9.2.1 Molecular Interactions
is the Debye screening length, with
being the ionic strength and
,
the distance between molecules,
is the size of the molecule,
is the charge number of the molecule,
is the Bjerrum length,
is the Boltzmann constant,
is the absolute temperature and
is the Avogadro number.
). Indeed, entanglement concentration (
) is in the range
for neutral polymers and polyelectrolytes in high salt concentration. If entanglement of a typical mucin (MW ∼250–1000 kDa) is considered then expected
range should be
mg/ml, which is typical for mucus gels but much lower than in serous mucus secretions like saliva and tears. At high concentrations, the overlap concentration of the side chains of the comb subunits can also be observed. If a cylindrical approximation for the shape of the oligosaccharide side chain is assumed then the range of brush overlap concentrations of a typical mucin is
mg/ml, and hence within the range of entanglement concentrations. It is, therefore, advantageous to use comb-brush (or bottle brush) polymers for mucoadhesive applications, when other mechanisms cannot be fully exploited and there is a need to maximise the entanglement effect.
9.2.2 Colloidal Interactions
9.2.2.1 Size and Ion Exclusion Filter
9.2.2.2 Contact Mechanics and Wettability
9.2.3 Dynamic Aspects
9.2.4 Goldilock’s Principle in Mucoadhesion
9.3 Experimental Approaches to Measuring Mucosal Interactions
Instrumental Technique
Physical Principle
Physical/Chemical Insights Key for Mucoadhesion
References (Mucoadhesive System/Ingredient)
Changes in light polarisation due to changes in thickness and refractive index of the adsorbed layer
Surface Plasmon Resonance Spectroscopy (SPR)
Changes in the plasmon coupling angle on a metal film (commonly gold) modified with adsorbed molecules
Dual Polarisation Interferometry (DPI)
Changes in the 2D interference pattern between two wave-guided beams due to adsorption of the material on one of them.
[35a] ·mucin adsorption
Optical Waveguide Light-mode Spectroscopy (OWLS)
Changes in the wave-mode coupling angle of a micrograting waveguide due to adsorption of the material
[36] ·cell adhesion to mucin
Quartz Crystal Microbalance, QCM-D (with Dissipation)
Changes in the resonance frequency and frequency of overtones (including higher overtones) and the rate of oscillation decay of a quartz microbalance crystal due to adsorption
Atomic Force Microscopy (AFM)
Imaging of molecules based on the interaction of AFM micro-fabricated tip and adsorbed materials
Confocal Scanning Laser Microscopy (CSLM)
Imaging of fluorescently labelled materials in a confocal plane
AFM Force Spectroscopy
Measurements of normal and shear forces between AFM tip or a colloidal particles and a flat surface.
Surface Force Apparatus (SFA)/Surface Force Balance (SFB)
Measurements of normal and shear forces between two flat surfaces (usually atomically flat e.g. using mica)
Absorption of infrared light of specific frequencies due to resonance with molecular vibrational modes
1H and 13C NMR
Changes in chemical shifts associated with binding
Isothermal Titration Calorimetry (ITC)
Compensation calorimetry of binding events during titration process
Dielectric Spectroscopy, Electrochemical Impedance Spectroscopy (EIS)
Changes in conductance and electrical impedance of adsorbed layers
9.3.1 Measuring Adhesion on the Molecular Level
and, to a lesser extent,
NMR [40] enable detailed characterisation of interaction of mucoadhesive material with mucosa. However, it is not uncommon that spectral interpretation is very challenging, unless small molecular weight model molecules are used to imitate the mucosa and mucoadhesive. However, despite ubiquitous use of surface characterisation and spectroscopic techniques, they provide only indirect information about intermolecular forces involved in mucoadhesion.
9.3.2 Tribology of Mucoadhesive Contacts
9.3.3 Macroscopic Methods
9.3.4 In Vivo Methodologies
9.4 Integrative Approaches. Layer-by-Layer Assembled Multilayers: A Tool for Studying Mucoadhesion
9.4.1 The Aims of the Integrative Approach
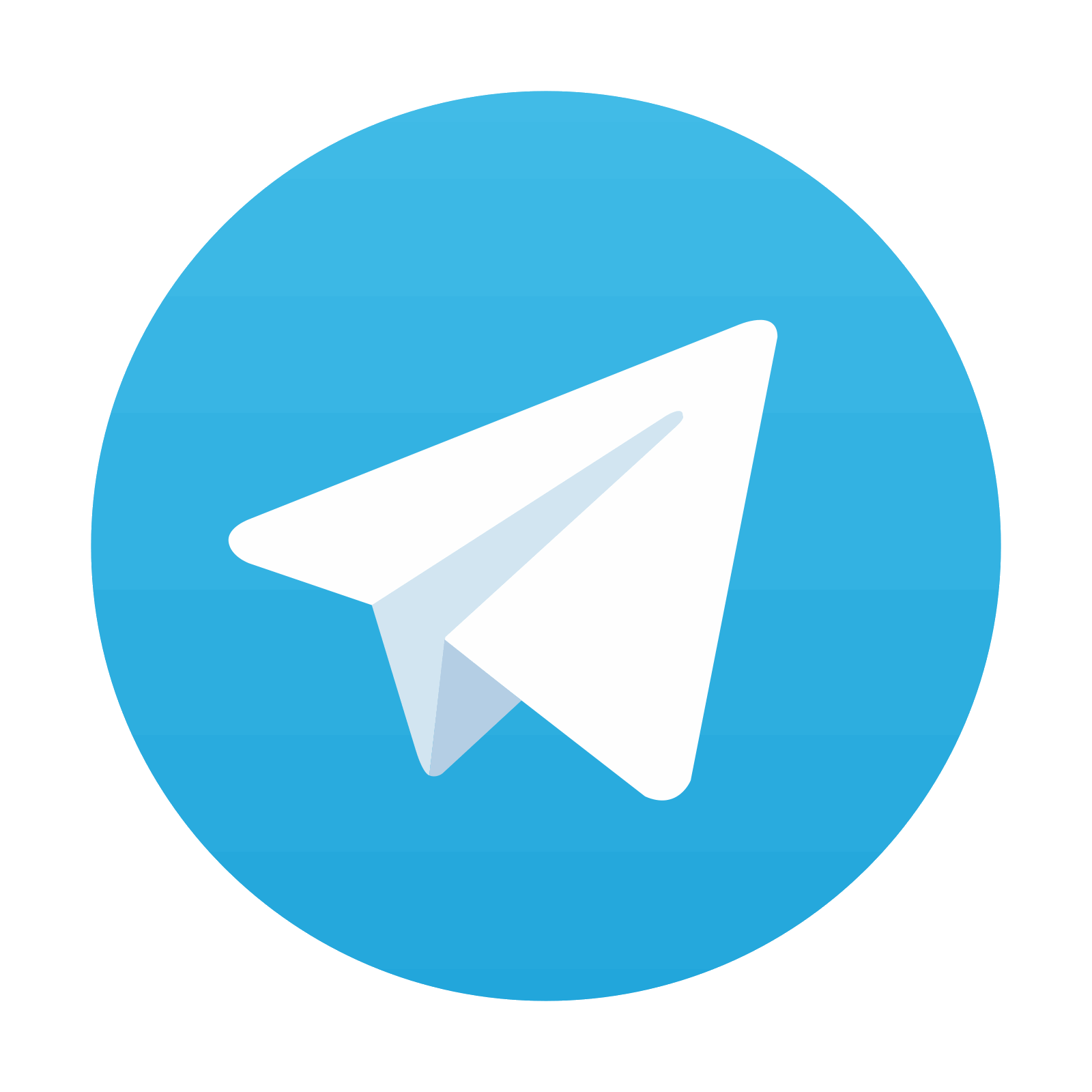
Stay updated, free articles. Join our Telegram channel
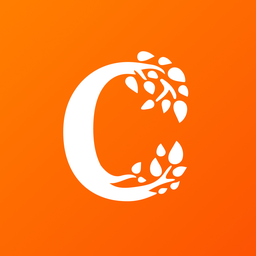
Full access? Get Clinical Tree
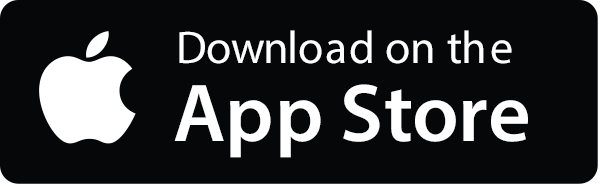
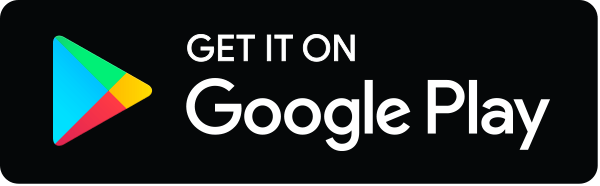