Fig. 3.1
Common spine anatomy. Note the anterior/posterior curvature typical of a normal, healthy spine (Reprinted from Ref. [1]. Copyright 2011 with permission from Suanders Elsevier)
The basic anatomic building blocks are the vertebrae, each numbered in a given region. Anteriorly, from the second cervical to the first sacral vertebrae, intervening disks act as a shock absorber and allow motion. A motion segment is therefore defined as the vertebral body above and below with the intervening disk (Fig. 3.2). The basic bone anatomy includes a large mass of bone, which makes up the anterior column support. Two bridges of bone protrude posteriorly to connect the anterior structures to the posterior structures. These are known as the pedicles. Posteriorly, two joints are formed from processes extending from the vertebrae above and the vertebrae below the disk. This articulation is known as the facet joint. The pedicles and articular processes are confluent with a sheet of bone that overlies the spinal cord/nerves called the lamina. Finally, the posteriormost portion of the bone is the spinous process, which serves as a point of attachment for muscle and ligaments.
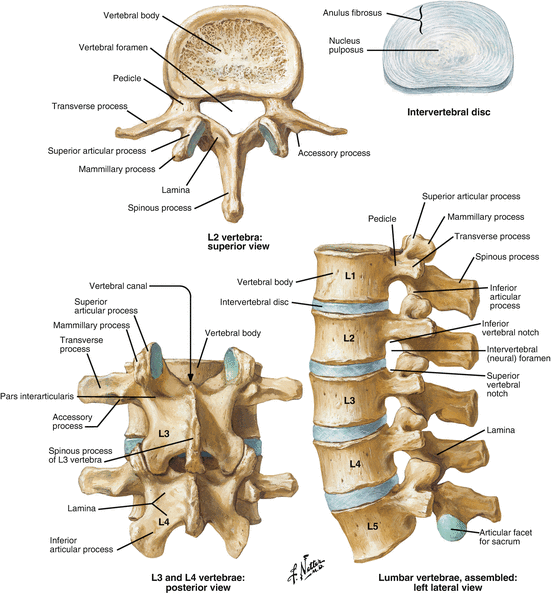
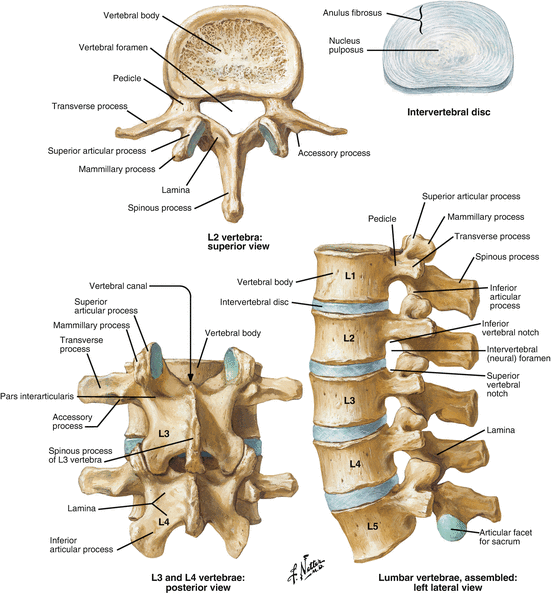
Fig. 3.2
Anatomy of the vertebra (Reprinted from Ref. [1] Copyright 2011 with permission from Suanders Elsevier)
The cervical spine is formed by seven vertebrae and includes the articulation of the skull with the spinal column. The first and second vertebrae (axis and atlas, respectively) have a unique structure that allows for articulation with the base of the skull with a smooth transition into the spinal column. These levels are also responsible for a large portion of the rotation and flexion/extension motions of the cervical spine. Their unique shape, location, and surrounding anatomy pose particular difficulties with surgical management; however, that is beyond the scope of this chapter. The lower portion of the cervical spine (subaxial region) consists of the C3–C7 vertebrae, which have a common structure similar to that described above. The thoracic spine is characterized by the addition of articulations for the ribs that are also attached to the sternum. This creates a significantly more rigid biologic construct with little motion through the thoracic region. The lumbar spine is again a mobile region of the spinal column and necessarily bears the brunt of the body load in an upright species such as humans. The sacrum then serves as the attachment point of the spinal column with the pelvis at the sacroiliac joints, and the coccyx is a vestigial tail remnant without structural implications. The sacrum and the coccyx are formed by the fusion of vertebrae, five in the sacrum and four in the coccyx.
3.2 Clinical Correlates
There are several clinical correlates with the use of metals in spinal surgery, but the most common is for the purposes of spinal fusion. With various disease processes including fracture, arthritis, and spinal deformity, the mainstay of treatment often involves fusion across several motion segments of the spine. The ultimate goal is to create a biologic fusion with bone growth across the fused segments, but this is not easily accomplished in a mobile spine. Unlike a long bone fracture, the natural state of spine segments is motion rather than rigid fusion. General bone growth characteristics require a relatively stable environment to allow bone growth and maturation similar to casting a long bone fracture. Conversely, as is seen in long bone fractures, instability and motion lead to excess bone formation without bridging the fracture. This creates a need for relative stability during the initial attempt at fusion and has been one of the main focuses in the use of metals with spine surgery.
Initial fixations consisted of wiring of spinous processes. As previously discussed, a motion segment consists of two vertebral bodies and the intervertebral disk. Prior to many of the advances in spine instrumentation, fusions were attempted without internal stabilization. While there is still a debate in the literature as to the role of instrumentation in spine fusions for certain degenerative processes, stabilization of the spine in cases of fracture, infection, instability, and deformity is ultimately a necessary part of the surgical management. Early techniques, such as wiring of the spinous processes first described in the literature by Dr. Berthold Ernest Hadra in 1891, served as the introductions of metals in spine surgery (Fig. 3.3) [8]. Over time, techniques and implants were modified to include rod systems, metal hooks, variously placed screws, and eventually pedicle screws, which are now one of the most commonly used implants in spine surgery today. The combination of pedicle screws with rods allows the surgeon to create a fairly rigid construct for the maintenance of spinal stability. Further, this has allowed for the correction of spinal deformities to an even greater extent. However, it has also introduced issues for consideration based on an individual basis.
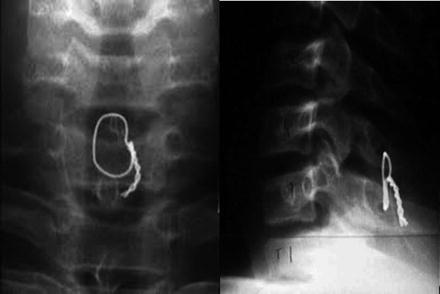
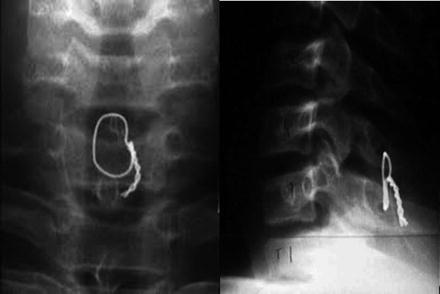
Fig. 3.3
Spinal fusion technique as described by Hadra http://www.wheelessonline.com/ortho/facet_joint_injuries
Several factors have influenced the metals used and the manner in which they have been implemented. Among these include the quality of the bone being manipulated, the intended use for the implants, and the patient-specific qualities such as age, surgical procedure, and outcome goals. While other surgical considerations are used to implement pedicle screw and rod constructs, such as anterior support with cages, we will focus on the specifics of metal implantation of screw and rod constructs.
Current techniques for spine fusion include the use of screws placed from the posterior through the pedicle (bridge of bone previously described) and into the vertebral body as shown in Fig. 3.4. Common metal alloys recognized by the governing agency for medical devices in the US Federal Drug Agency (FDA) for use in the production of pedicle screws include 316L stainless steel, 316LVM stainless steel, 22Cr-13Ni-5Mn stainless steel, Ti-6Al-4V, and unalloyed titanium (FDA final rule: Pedicle Screw Systems – 21 Code of Federal Regulations Part 888.3070). Nitinol, or NiTi, has also been evaluated for use more recently because its modulus of elasticity can be more closely matched to the bone and because of improved bone ingrowth when produced in a porous form [9, 10]. These advantages have not been fully parsed out in a clinical setting. Similar metals are often used to create the rods used in these systems with the inclusion of CoCr alloy (CoCr) as well. The ultimate goal for the pedicle screw is a metal or metal alloy that interfaces with the bone to allow ongrowth/ingrowth of the screw for improved screw purchase. This in turn reduces the incidence of screw loosening, screw pullout, or failure of the screw cyclic loading over time. Similarly, the rod is used in combination with screws to correct a deformity and/or stabilize a segment of the spine over time to allow a biologic bone fusion to occur. The current use of these metal implants is a time-sensitive process in which there is a race between ultimate implant failure and biologic healing. While this is ever improving, continued advances may help to mitigate failures of the bone and or the metal over time.
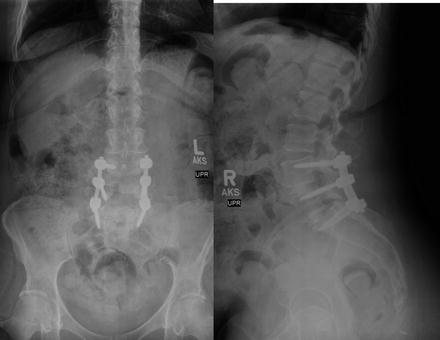
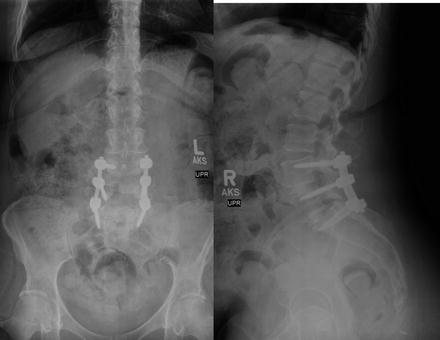
Fig. 3.4
Typical placement of spinal instrumentation including pedicle screws and rods
In situ, this race is partially dependent on the quality of the bone. In certain pediatric disease processes, as well as adults with osteopenia/osteoporosis, the loss of bone structure decreases the purchase of the screws and can lead to screw failure over time. This is seen clinically with screw pullout, fractures through instrumented segments, loss of deformity correction, and proximal fractures above a stiff construct. Currently, one of the most common practices is to augment the vertebral bone with a cement through which the screw may be inserted and provide improved purchase. Ultimately, the bone quality, in part, drives the surgical planning and choice of metal implants. The placement of a titanium alloy may be preferred to the stiffer metals/alloys to reduce the stress produced at the construct-bone interface. Also, less correction may be expected with these deformities with the ultimate goal of a solid bony fusion in mind (Fig. 3.5).
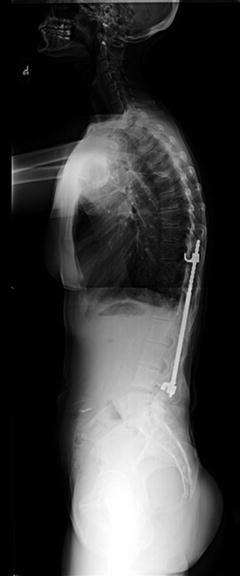
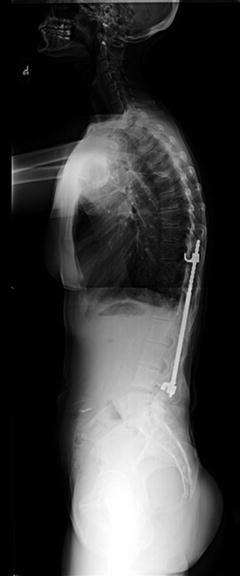
Fig. 3.5
Harrington rods in a patient with spinal fusion, one of the first devices to stabilize the spine and correct deformity based on distraction
When bone quality is less of an issue, such as younger patients, and the metal implants may be selected to allow for the greater correction of spine deformities, several issues still exist. One such issue inherent to the surgery is the inability to have a custom-contoured rod. Typically, a straight or pre-bent rod is contoured intraoperatively to fit the patient profile. This can cause several issues with the integrity of the metal as described in further detail later in the chapter. Also, over time, these rods have been shown to lose some of the contour in situ and, as a result, decrease the amount of surgical correction. Ultimately, the best scenario would involve the placement of a rod pre-contoured to the expected final position of the spine.
In the face of pedicle screw and rod instrumentation, advantages and disadvantages to the use of various metal/metal alloys have been studied and are further described. Clinically, the ultimate goal of instrumentation is to provide fixation that allows stability over time and can withstand the body’s environment in the face of cyclic loading. This has improved over time with changing surgical techniques and the improved understanding of metals and alloys. However, there is continued room for improvement. We will continue with a more in-depth discussion of the various metals and metal alloys commonly seen in spinal instrumentation with perceived advantages and disadvantages (Fig. 3.6).
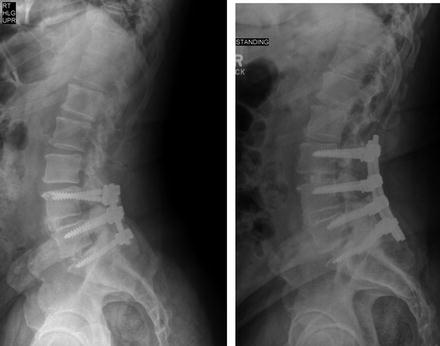
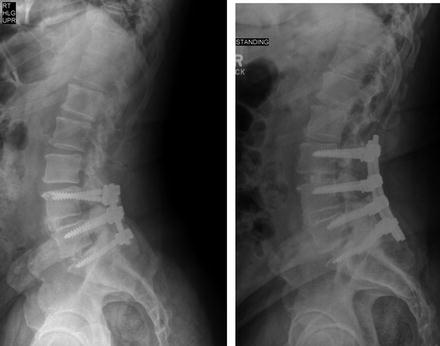
Fig. 3.6
Fractured pedicle screw in the S1 vertebra
3.3 Ferrous Alloys
Ferrous alloys encompass all metal alloys that use iron (Fe) as its predominant component. These are also commonly referred to as steels. The predominant orthopedic steel is termed A316L (AISI designation) stainless steel. However, there are other A316 alloys such as AISI A136Cb with compositions tailored to reduce grain boundary chromium carbide formation at elevated temperatures. These alloys retain the austenitic face-centered (FCC) unit cell structure, hence the “A” prefix in the designation. This structure enables ease of forming, high ductility (increased formability), and high impact strength while being nonmagnetic (allows for a patient to undergo magnetic resonance imaging, MRI). The FCC structure is retained at low temperatures (below the eutectoid) because the significant amount of nickel creates a substitutional solid solution at temperatures above the eutectoid. The 316 series stainless steel is a modified 304 stainless steel with increased Mo to reduce pitting corrosion and reduced carbon to decrease the sensitivity of the alloy to forming undesired carbides in the grain boundaries, increasing its weldability. The additions of chromium (between 16 and 18 wt%) and molybdenum (2–3 wt%) enhance the corrosion resistance through the formation of oxides on the surface.
A316 alloys are also easily and economically processed. They are readily manufactured in existing basic oxygen furnaces in high volumes. Stainless steels, in general, lend themselves to forging processes, machining, and welding to create a specified device. They can be cold-worked, such as drawing and bending without necessary annealing, allowing for economic manufacture of spinal instrumentation.
3.4 Titanium Alloys
Titanium and its alloys offer unique advantages over steels in medicine, predominantly, low weight and high corrosion resistance. While there are 38 grades of titanium and its alloys, approximately five of those grades constitute almost 80 % of the production in the USA and a majority of that is Ti6Al4V (ASTM Grade 5; Type III) [11]. For the purposes of this chapter, it is easier to consider the available biomedical titanium not from their compositions, such as with steel, but by their microstructure.
First are the α-type titanium alloys. These are generally the commercially pure (CP Ti) forms but there are alloys as well. The α-phase in titanium is characterized by the hexagonal close-packed (HCP) unit cell. These alloys are characterized by good strength, toughness, creep resistance, and weldability. Common alloying elements are aluminum and tin. Other α-phase stabilizers are O and N. Strengthening is accomplished through increasing oxygen concentrations, from 0.18 wt% for ASTM Grade 1 CP Ti (240 MPa tensile strength) to 0.40 wt% for Grade 4 CP Ti (550 MPa tensile strength); however, this comes at a cost of decreasing formability.
Second are the (α + β)-type titanium alloys. The most common is Ti6Al4V. (α + β) describes the mixed microstructure where, in general, the “rigid” α-phase HCP unit cell is mixed with a more ductile β-phase, which has a BCC unit cell. In the case of Ti6AL4V, vanadium stabilizes the high-temperature β-phase to increase alloy ductility as well as to increase its strength well beyond the single-phase alloys. Additionally, these alloys can be strengthened via post-synthesis heat treatments rather than composition, reducing production costs and material complexity.
The β-phase enables super-plastic behavior of (α + β)-type titanium alloys. The mechanism of this comes from a combination of high diffusivity in the β-phase combined with grain coarsening in the α-phase [11]. The implications are that without any special processing Ti6Al4V can undergo an elongation of 750–1,100 % at elevated temperatures, further enhancing the formability of Ti6Al4V to generate medical devices. Additionally, these phenomena allow the surgeon to contour Ti6Al4V spinal instrumentation at room temperature to match larger deformities in vivo. However, both springback and the Bauschinger effect cannot be neglected as that can lead to pedicle screw pullout and subsequent instrumentation failure.
β-type titanium alloys are becoming more popular in orthopedic applications as their lower modulus is believed to reduce the effect of stress-shielding on the bone as well as exhibit an increased fracture toughness as compared to an alpha-beta alloy of equivalent aging. Generally, β-type titanium alloys have good ductility, relatively low strength, low modulus, and excellent uniaxial formability. The ability to harden the alloy is easily controlled during the cooling process enabling the alloy to be better designed to the spinal biomechanical environment. These properties also suggest β-type titanium alloys as effective metals in spinal applications given the metal can be formed at room temperature without appreciable cold-work hardening. The more common biomedical alloy in this form is TNTZ (titanium-niobium-tantalum-zirconium) where each alloying element is both a beta-phase stabilizer and biocompatible.
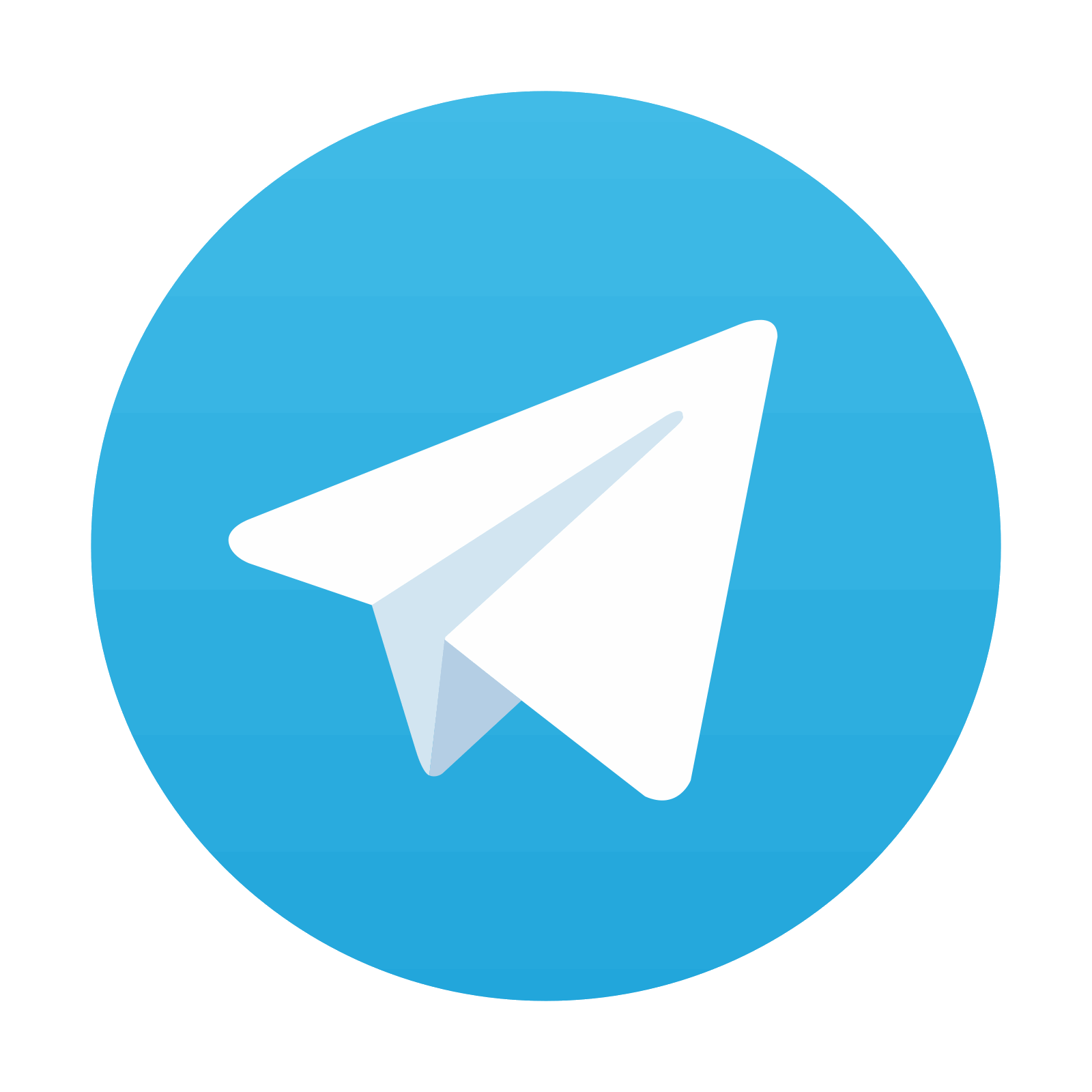
Stay updated, free articles. Join our Telegram channel
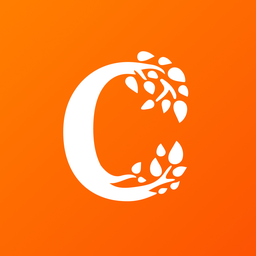
Full access? Get Clinical Tree
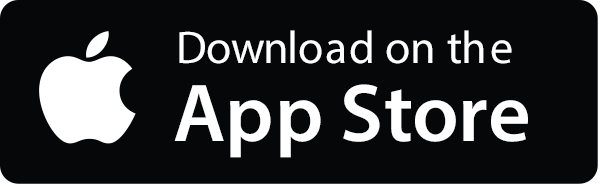
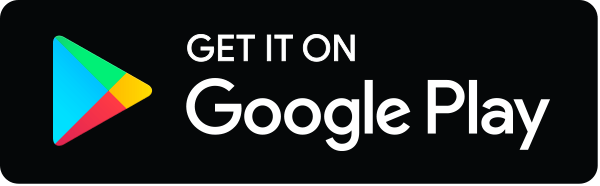