Objectives
- Describes the generation of a pressure gradient between the atmosphere and the alveoli.
- Describes the passive expansion and recoil of the alveoli.
- Defines the mechanical interaction of the lung and the chest wall, and relates this concept to the negative intrapleural pressure.
- Describes the pressure-volume characteristics of the lung and the chest wall, and predicts changes in the compliance of the lung and the chest wall in different physiologic and pathologic conditions.
- States the roles of pulmonary surfactant and alveolar interdependence in the recoil and expansion of the lung.
- Defines the functional residual capacity (FRC), and uses his or her understanding of lung-chest wall interactions to predict changes in FRC in different physiologic and pathologic conditions.
- Defines airways resistance and lists the factors that contribute to or alter the resistance to airflow.
- Describes the dynamic compression of airways during a forced expiration.
- Relates changes in the dynamic compliance of the lung to alterations in airways resistance.
- Lists the factors that contribute to the work of breathing.
- Predicts alterations in the work of breathing in different physiologic and pathologic states.
Mechanics of Breathing: Introduction
Air, like other fluids, moves from a region of higher pressure to one of lower pressure. Therefore, for air to be moved into or out of the lungs, a pressure difference between the atmosphere and the alveoli must be established. If there is no pressure difference, no airflow will occur.
Under normal circumstances, inspiration is accomplished by causing alveolar pressure to fall below atmospheric pressure. When the mechanics of breathing are being discussed, atmospheric pressure is conventionally referred to as 0 cm H2O, so lowering alveolar pressure below atmospheric pressure is known as negative-pressure breathing. As soon as a pressure difference sufficient to overcome the resistance to airflow offered by the conducting airways is established between the atmosphere and the alveoli, air flows into the lungs. It is also possible to cause air to flow into the lungs by raising the pressure at the nose and mouth above alveolar pressure. This positive-pressure ventilation is generally used on patients unable to generate a sufficient pressure difference between the atmosphere and the alveoli by normal negative-pressure breathing. Air flows out of the lungs when alveolar pressure is sufficiently greater than atmospheric pressure to overcome the resistance to airflow offered by the conducting airways.
Generation of a Pressure Difference between Atmosphere and Alveoli
During normal negative-pressure breathing, alveolar pressure is made lower than atmospheric pressure. This is accomplished by causing the muscles of inspiration to contract, which increases the volume of the alveoli, thus lowering the alveolar pressure according to Boyle law. (See Appendix II: The Laws Governing the Behavior of Gases.)
The alveoli are not capable of expanding themselves. They only expand passively in response to an increased distending pressure across the alveolar wall. This increased transmural pressure difference, generated by the muscles of inspiration, further opens the highly distensible alveoli, and thus lowers the alveolar pressure. The transmural pressure difference is conventionally calculated by subtracting the outside pressure (in this case, the intrapleural pressure) from the inside pressure (in this case, the alveolar pressure).
The pressure in the thin space between the visceral and parietal pleura is normally slightly subatmospheric, even when no inspiratory muscles are contracting. This negative intrapleural pressure (sometimes also referred to as negative intrathoracic pressure) of –3 to –5 cm H2O is mainly caused by the mechanical interaction between the lung and the chest wall. At the end of expiration, when all the respiratory muscles are relaxed, the lung and the chest wall are acting on each other in opposite directions. The lung is tending to decrease its volume because of the inward elastic recoil of the distended alveolar walls; the chest wall is tending to increase its volume because of its outward elastic recoil. Thus, the chest wall is acting to hold the alveoli open in opposition to their elastic recoil. Similarly, the lung is acting by its elastic recoil to hold the chest wall in.
Because of this interaction, the pressure is negative at the surface of the very thin (about 10–30 μm in thickness at normal lung volumes), fluid-filled pleural space, as seen on the left in Figure 2–1. There is normally no gas in the intrapleural space, and the lung is held against the chest wall by the thin layer of serous intrapleural liquid, estimated to have a total volume of about 15 to 25 mL in an average adult.
Figure 2–1.
Representation of the interaction of the lung and chest wall. Left: At end expiration, the muscles of respiration are relaxed. The inward elastic recoil of the lung is balanced by the outward elastic recoil of the chest wall. Intrapleural pressure is −5 cm H2O; alveolar pressure is 0. The transmural pressure difference across the alveolus is therefore 0 cm H2O −(−5 cm H2O), or 5 cm H2O. Since alveolar pressure is equal to atmospheric pressure, no airflow occurs. Right: During inspiration, contraction of the muscles of inspiration causes intrapleural pressure to become more negative. The transmural pressure difference increases and the alveoli are distended, decreasing alveolar pressure below atmospheric pressure, which causes air to flow into the alveoli.
Initially, before any airflow occurs, the pressure inside the alveoli is the same as atmospheric pressure—by convention 0 cm H2O. Alveolar pressure is greater than intrapleural pressure because it represents the sum of the intrapleural pressure plus the alveolar elastic recoil pressure:
Alveolar pressure = intrapleural pressure + alveolar elastic recoil pressure
The muscles of inspiration act to increase the volume of the thoracic cavity. The outside of the lung (the visceral pleura) adheres to the inside of the chest wall (the parietal pleura). As the inspiratory muscles contract, expanding the thoracic volume and increasing the outward stress on the lung, the intrapleural pressure becomes more negative. Therefore, the transmural pressure difference tending to distend the alveolar wall (sometimes called the transpulmonary pressure) increases as shown in Figure 2–1, and the alveoli enlarge passively. Increasing alveolar volume lowers alveolar pressure and establishes the pressure gradient for airflow into the lung. In reality, only a small percentage of the total number of alveoli are directly exposed to the intrapleural surface pressure, and at first thought, it is difficult to see how alveoli located centrally in the lung could be expanded by a more negative intrapleural pressure. However, analysis has shown that the pressure at the pleural surface is transmitted through the alveolar walls to more centrally located alveoli and small airways. This structural interdependence of alveolar units is depicted in Figure 2–2.
Figure 2–2.
Structural interdependence of alveolar units. The pressure difference across the outermost alveoli is transmitted mechanically through the lung via the alveolar septa. The insets show the author’s idea of what might happen in negative-pressure breathing and positive-pressure ventilation. In negative-pressure breathing (Inset A) the mechanical stress would likely be transmitted from the more exterior alveoli (those closest to the chest wall) to more interior alveoli, so the exterior alveoli might be more distended. In positive-pressure ventilation (Inset B) the lungs must push against the diaphragm and rib cage to move them. The outermost alveoli might be more compressed than those located more interiorly.
Note that in Figure 2–1, the inward alveolar elastic recoil calculated by the equation above is equal to the transmural pressure difference. This is true under static conditions, but they may differ slightly during a breath as the alveoli are stretched.
The muscles of inspiration include the diaphragm, the external intercostals, and the accessory muscles of inspiration, which include the sternocleidomastoid, the trapezius, and the muscles of the vertebral column.
The diaphragm is a large (about 250 cm2 in surface area) dome-shaped muscle that separates the thorax from the abdominal cavity. As mentioned in Chapter 1, the diaphragm is considered to be an integral part of the chest wall and must always be considered in the analysis of chest wall mechanics.
The diaphragm is the primary muscle of inspiration. When a person is in the supine position, the diaphragm is responsible for about two thirds of the air that enters the lungs during normal quiet breathing (which is called eupnea). (When a person is standing or seated in an upright posture, the diaphragm is responsible for only about one third to one half of the tidal volume.) It is innervated by the 2 phrenic nerves, which leave the spinal cord at the third through the fifth cervical segments.
The muscle fibers of the diaphragm are inserted into the sternum and the 6 lower ribs and into the vertebral column by the two crura. The other ends of these muscle fibers converge to attach to the fibrous central tendon, which is also attached to the pericardium on its upper surface (Figure 2–3). During normal quiet breathing, contraction of the diaphragm causes its dome to descend 1 to 2 cm into the abdominal cavity, with little change in its shape. This elongates the thorax and increases its volume. These small downward movements of the diaphragm are possible because the abdominal viscera can push out against the relatively compliant abdominal wall. During a deep inspiration, the diaphragm can descend as much as 10 cm. With such a deep inspiration, the limit of the compliance of the abdominal wall is reached, abdominal pressure increases, and the indistensible central tendon becomes fixed against the abdominal contents. After this point, contraction of the diaphragm against the fixed central tendon elevates the lower ribs (Figure 2–3).
If one of the leaflets of the diaphragm is paralyzed (eg, because of transection of one of the phrenic nerves), it will “paradoxically” move up into the thorax as intrapleural pressure becomes more negative during a rapid inspiratory effort.
When they are stimulated to contract, the external intercostal, parasternal intercostal, and scalene muscles raise and enlarge the rib cage. The parasternal muscles, which are usually considered part of the internal intercostals, are inspiratory muscles and may be partly responsible for raising the lower ribs. The scalene muscles appear to contract in normal quiet breathing and are therefore not accessory muscles. Figure 2–4 demonstrates how contraction of these muscles increases the anteroposterior dimension of the chest as the ribs rotate upward about their axes and also increases the transverse dimension of the lower portion of the chest. These muscles are innervated by nerves leaving the spinal cord at the first through the 11th thoracic segments. During inspiration, the diaphragm and inspiratory rib cage muscles contract simultaneously. If the diaphragm contracted alone, the rib cage muscles would be pulled inward (this is called retraction). If the inspiratory muscles of the rib cage contracted alone, the diaphragm would be pulled upward into the thorax.
Figure 2–4.
Illustration of the actions of contraction of the intercostal muscles, abdominal muscles, and accessory muscles. (Reprinted with permission of the publisher from Weibel ER. The Pathway for Oxygen. Cambridge, MA: Harvard University Press; 1984:304. Copyright © 1984 by the President and Fellows of Harvard College.)
The accessory muscles of inspiration are not involved during normal quiet breathing but may be called into play during exercise; during the inspiratory phase of coughing or sneezing; or in a pathologic state, such as asthma. For example, the sternocleidomastoid elevates the sternum and helps increase the anteroposterior and transverse dimensions of the chest. Dyspnea, the feeling that breathing is difficult, may sometimes result from fatigue of the inspiratory muscles. Other potential causes of dyspnea will be discussed in Chapter 9.
Expiration is passive during normal quiet breathing, and no respiratory muscles contract. As the inspiratory muscles relax, the increased elastic recoil of the distended alveoli is sufficient to decrease the alveolar volume and raise alveolar pressure above atmospheric pressure. Now the pressure gradient for airflow out of the lung has been established.
Although the diaphragm is usually considered to be completely relaxed during expiration, it is likely that some diaphragmatic muscle tone is maintained, especially when one is in the horizontal position. The inspiratory muscles may also continue to contract actively during the early part of expiration, especially in obese people. This so-called braking action may help maintain a smooth transition between inspiration and expiration. It may also be important during speech production.
Active expiration occurs during exercise, speech, singing, the expiratory phase of coughing or sneezing, and in pathologic states such as chronic bronchitis. The main muscles of expiration are the muscles of the abdominal wall, including the rectus abdominis, the external and internal oblique muscles, the transversus abdominis, and the internal intercostal muscles.
When the abdominal muscles contract, they increase abdominal pressure and push the abdominal contents against the relaxed diaphragm, forcing it upward into the thoracic cavity. They also help depress the lower ribs and pull down the anterior part of the lower chest.
Contraction of the internal intercostal muscles depresses the rib cage downward in a manner opposite to the actions of the external intercostals.
The events occurring during the course of an idealized normal quiet breath, which are summarized in Table 2–1, are shown in Figure 2–5. For the purpose of clarity, inspiration, and expiration are considered to be of equal duration, although during normal quiet breathing, the expiratory phase is 2 to 3 times longer than the inspiratory phase, that is, the normal I:E ratio is 1:2 to 1:4 in eupneic breathing.
Inspiration |
|
Expiration (Passive) |
|
Figure 2–5.
Volume, pressure, and airflow changes during a single idealized eupneic respiratory cycle. Inspiration is on the left side, expiration on the right. Described in text. (Reproduced with permission from Comroe. Physiology of Respiration. 2nd ed. Chicago: Year Book Medical Publishers; 1974.)
The volume of air entering or leaving the lungs can be measured with a spirometer, as will be described in Chapter 3 (Figure 3–4). Airflow can be measured by breathing through a pneumotachograph, which measures the instantaneous pressure difference across a fixed resistance. The intrapleural pressure can be estimated by having a subject swallow a balloon into the intrathoracic portion of the esophagus. The pressure then measured in the balloon is nearly equal to intrapleural pressure. Alveolar pressures are not directly measurable and must be calculated.
Initially, alveolar pressure equals atmospheric pressure, and so no air flows into the lung. Intrapleural pressure is –5 cm H2O. Contraction of the inspiratory muscles causes intrapleural pressure to become more negative as the lungs are pulled open and the alveoli are distended. Note the 2 different courses for changes in intrapleural pressure. The dashed line (which would not really be straight for reasons discussed in the next section) predicts the changes in intrapleural pressure necessary to overcome the elastic recoil of the alveoli. The solid line is a more accurate representation of intrapleural pressure because it also includes the additional pressure work that must be done to overcome the resistance to airflow and tissue resistance discussed later in this chapter. As the alveoli are distended, the pressure inside them falls below atmospheric pressure and air flows into the alveoli, as seen in the tidal volume panel. As the air flows into the alveoli, alveolar pressure returns to 0 cm H2O and airflow into the lung ceases. At the vertical line (at 2 seconds), the inspiratory effort ceases and the inspiratory muscles relax. Intrapleural pressure becomes less negative, and the elastic recoil of the alveolar walls (which is increased at the higher lung volume) compresses the alveolar gas. This raises alveolar pressure above atmospheric pressure so that air flows out of the lung until an alveolar pressure of 0 cm H2O is restored. At this point, airflow ceases until the next inspiratory effort.
Pressure-Volume Relationships in the Respiratory System
The relationship between changes in the pressure distending the alveoli and changes in lung volume is important to understand because it dictates how easily the lung inflates with each breath. As mentioned before, the alveolar-distending pressure is often referred to as the transpulmonary pressure. Strictly speaking, the transpulmonary pressure is equal to the pressure in the trachea minus the intrapleural pressure. Thus, it is the pressure difference across the whole lung. However, the pressure in the alveoli is the same as the pressure in the airways—including the trachea—at the beginning or end of each normal breath, that is, end-expiratory or end-inspiratory alveolar pressure is 0 cm H2O (Figure 2–5). Therefore, at the beginning or end of each lung inflation, alveolar-distending pressure can be referred to as the transpulmonary pressure.
The pressure-volume characteristics of the lung can be inspected in several ways. One of the simplest is to remove the lungs from an animal or a cadaver and then graph the changes in volume that occur for each change in transpulmonary pressure the lungs are subjected to (Figure 2–6).
Figure 2–6 shows that as the transpulmonary pressure increases, the lung volume increases. This relationship is not a straight line: The lung is composed of living tissue, and although the lung distends easily at low lung volumes, at high lung volumes the distensible components of alveolar walls have already been stretched, and large increases in transpulmonary pressure yield only small increases in volume.
The slope between 2 points on a pressure-volume curve is known as the compliance. Compliance is defined as the change in volume divided by the change in pressure. Lungs with high compliance have a steep slope on their pressure-volume curves; that is, a small change in distending pressure will cause a large change in volume. It is important to remember that compliance is the inverse of elastance, elasticity, or elastic recoil. Compliance denotes the ease with which something can be stretched or distorted; elastance refers to the tendency for something to oppose stretch or distortion, as well as to its ability to return to its original configuration after the distorting force is removed.
There are several other interesting things to note about an experiment like that illustrated in Figure 2–6. The curve obtained is the same whether the lungs are inflated with positive pressure (by forcing air into the trachea) or with negative pressure (by suspending the lung, except for the trachea, in a closed chamber and pumping out the air around the lung). So when the lung alone is considered, only the transpulmonary pressure is important, not how the transpulmonary pressure is generated. As was seen in Figure 2–2A and B, when the lungs and chest wall are considered together, generation of the transmural pressure difference by positive pressure does have effects different from generation of the transmural pressure difference by negative pressure. A second feature of the curve in Figure 2–6 is that there is a difference between the pressure-volume curve for inflation and the curve for deflation, as shown by the arrows. Such a difference is called hysteresis. One possible explanation for this hysteresis is the stretching on inspiration and the compression on expiration of the film of surfactant that lines the air-liquid interface in the alveoli (discussed later in this chapter). Surfactant has less effect on decreasing surface tension during inspiration than during expiration because of movement of surfactant molecules from the interior of the liquid phase to the surface during inspiration. Another explanation is that some alveoli or small airways may open on inspiration (“recruitment”) and close on expiration (“derecruitment”); the recruitment of collapsed alveoli or small airways requires energy and may be responsible for the lower inflection point seen on some inspiratory pressure-volume curves (see the air inflation curve in Figure 2–8). Finally, it is helpful to think of each alveolus as having its own pressure-volume curve like that shown in Figure 2–6, although some researchers believe that lung volume changes primarily by recruitment and derecruitment of alveoli rather than by volume changes of individual alveoli.
It may be surprising that there is no general agreement about what happens to alveoli as lung volume changes. As was shown in Figure 2–2, all alveoli could expand approximately equally during inspiration or some could expand more than others (eg, those closest to the pleura during negative-pressure breathing; those more centrally located during positive-pressure ventilation). As stated above, some researchers believe that most of the changes in lung volume during normal breathing occur by recruitment and derecruitment of unopened alveoli, with little change in volume of those already open, or by changes in the size of alveolar ducts with little change in alveolar volume at all. Whether alveoli expand by simply increasing the length of alveolar septae or opening folded areas or pleats is also not agreed upon.
The compliance of the lung and the chest wall provides very useful data for the clinical evaluation of a patient’s respiratory system because many diseases or pathologic states affect the compliance of the lung, of the chest wall, or both. The lung and the chest wall are physically in series with each other, and therefore their compliances add as reciprocals:
Conversely, the elastances of the lung and chest wall add directly.
Compliances in parallel add directly. Therefore, both lungs together are more compliant than either one alone; 2 alveoli in parallel are similarly more compliant than 1 alone.
To make clinical determinations of pulmonary compliance, one must be able to measure changes in pressure and in volume. Volume changes can be measured with a spirometer, but measuring pressure changes is more difficult because changes in the transmural pressure difference must be taken into account. For the lungs, the transmural pressure difference is transpulmonary pressure (alveolar minus intrapleural); for the chest wall, the transmural pressure difference is intrapleural pressure minus atmospheric pressure. As described previously, intrapleural pressure can be measured by having the patient swallow an esophageal balloon. The compliance curve for the lung can then be generated by having the patient take a very deep breath and exhale in stages, stopping periodically for pressure and volume determinations. During these determinations, no airflow is occurring; alveolar pressure therefore equals atmospheric pressure, 0 cm H2O. Similar measurements can be made as the patient inhales in stages from a low lung volume to a high lung volume. Such curves are called static compliance curves because all measurements are made when no airflow is occurring. The compliance of the chest wall is normally obtained by determining the compliance of the total system and the compliance of the lungs alone and then calculating the compliance of the chest wall according to the above formula. Dynamic compliance, in which pressure-volume characteristics during the breath are considered, will be discussed later in this chapter.
Representative static compliance curves for the lungs are shown in Figure 2–7. Note that these curves correspond to the expiratory curve in Figure 2–6. Many pathologic states shift the curve to the right (ie, for any increase in transpulmonary pressure there is less of an increase in lung volume). A proliferation of connective tissue called fibrosis may be seen in sarcoidosis or after chemical or thermal injury to the lungs. Such changes will make the lungs less compliant, or “stiffer,” and increase alveolar elastic recoil. Similarly, pulmonary vascular engorgement or areas of collapsed alveoli (atelectasis) also make the lung less compliant. Other conditions that interfere with the lung’s ability to expand (such as the presence of air, excess fluid, or blood in the intrapleural space) will effectively decrease the compliance of the lungs. Emphysema increases the compliance of the lungs because it destroys the alveolar septal tissue that normally opposes lung expansion.
The compliance of the chest wall is decreased in obese people, for whom moving the diaphragm downward and the rib cage up and out is much more difficult. People suffering from a musculoskeletal disorder that leads to decreased mobility of the rib cage, such as kyphoscoliosis, also have decreased chest wall compliance. Other conditions that can decrease the compliance of the chest wall include ossification of costal cartilage, skin scars from burn injuries, and abdominal distension.
Because they must generate greater transpulmonary pressures to breathe in the same volume of air, people with decreased compliance of the lungs must do more work to inspire than those with normal pulmonary compliance. Similarly, more muscular work must be done by someone with decreased chest wall compliance than by a person with normal chest wall compliance. In both cases, the person would be likely to breathe at a greater rate with a smaller tidal volume (the volume of air coming into and out of the respiratory system per breath).
As noted in the beginning of this section, lung compliance is volume-dependent. It is greater at low lung volumes and lower at high lung volumes. For this reason, the term specific compliance is often used to denote compliance with reference to the original lung volume.
The total compliance of a normal person near the normal end-expiratory lung volume (the functional residual capacity [FRC]) is about 0.1 L/cm H2O. The compliance of the lungs is about 0.2 L/cm H2O; that of the chest wall is also about 0.2 L/cm H2O.
The elastic recoil of the lungs is partly due to the elastic properties of the pulmonary parenchyma itself. Elastin is more compliant or distensible and is important at low or normal lung volumes. Collagen is less compliant or distensible and is not usually stressed until lung volume is large. However, there is another component of the elastic recoil of the lung besides the elastin, collagen, and other constituents of the lung tissue. That other component is the surface tension at the air-liquid interface in the alveoli.
Surface tension is a force that occurs at any gas-liquid interface (or even interfaces between 2 immiscible liquids) and is generated by the cohesive forces between the molecules of the liquid. These cohesive forces balance each other within the liquid phase but are unopposed at the surface of the liquid. Surface tension is what causes water to bead and form droplets. It causes a liquid to shrink to form the smallest possible surface area. The unit of measurement of surface tension is dynes per centimeter (dyn/cm).
The role of the surface tension forces in the elastic recoil of the lung can be demonstrated in an experiment such as that shown in Figure 2–8.
In this experiment, a pressure-volume curve for an excised lung was generated as was done in Figure 2–6. Because the lung was inflated with air, an air-liquid interface was present in the lung, and surface tension forces contributed to alveolar elastic recoil. Then, all the gas was removed from the lung, and it was inflated again, this time with saline instead of with air. In this situation, surface tension forces are absent because there is no air-liquid interface. The elastic recoil is due only to the elastic recoil of the lung tissue itself. Note that there is no hysteresis with saline inflation. Whatever causes the hysteresis appears to be related to surface tension in the lung. To recapitulate, the curve at left (saline inflation) represents the elastic recoil due to only the lung tissue itself. The curve at right demonstrates the recoil due to both the lung tissue and the surface tension forces. The difference between the 2 curves is the recoil due to surface tension forces.
The demonstration of the large role of surface tension forces in the recoil pressure of the lung led to consideration of how surface tension affects the alveoli. One traditional way of thinking about this has been to consider the alveolus to be a sphere hanging from the airway, as in Figure 2–9. The relationship between the pressure inside the alveolus and the wall tension of the alveolus would then be given by Laplace’s law (units in parentheses).
This can be rearranged as
The surface tension of most liquids (such as water) is constant and not dependent on the surface area of the air-liquid interface. Consider what this would mean in the lung, where alveoli of different sizes are connected to each other by common airways and collateral ventilation pathways (described in Chapter 1). If 2 alveoli of different sizes are connected by a common airway (Figure 2–10) and the surface tension of the 2 alveoli is equal, then according to Laplace’s law, the pressure in the small alveolus must be greater than that in the larger alveolus and the smaller alveolus will empty into the larger alveolus. If surface tension is independent of surface area, the smaller the alveolus on the right becomes, the higher the pressure in it.
Thus, if the lung were composed of interconnected alveoli of different sizes (which it is) with a constant surface tension at the air-liquid interface, it would be expected to be inherently unstable, with a tendency for smaller alveoli to collapse into larger ones. Normally, this is not the case, which is fortunate because collapsed alveoli require very great distending pressures to reopen, partly because of the cohesive forces at the liquid-liquid interface of collapsed alveoli. At least 2 factors cause the alveoli to be more stable than this prediction based on constant surface tension. The first factor is a substance called pulmonary surfactant, which is produced by specialized alveolar cells, and the second is the structural interdependence of the alveoli.
The surface tension of a liquid can be measured with an apparatus like that shown in Figure 2–11.
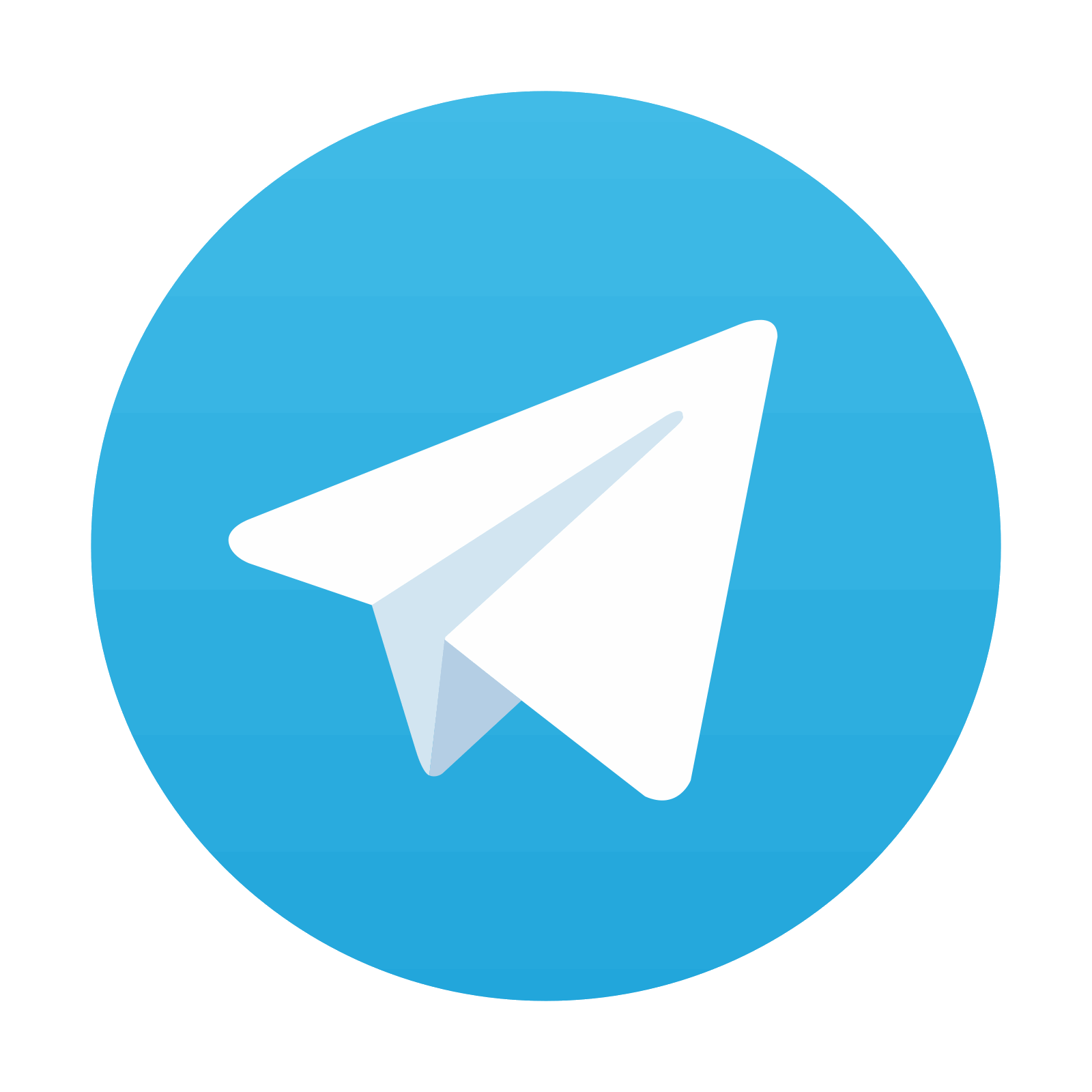
Stay updated, free articles. Join our Telegram channel
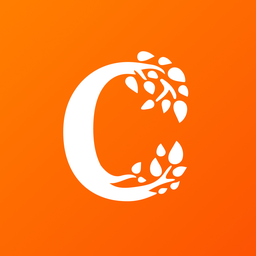
Full access? Get Clinical Tree
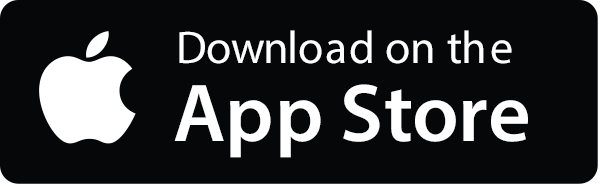
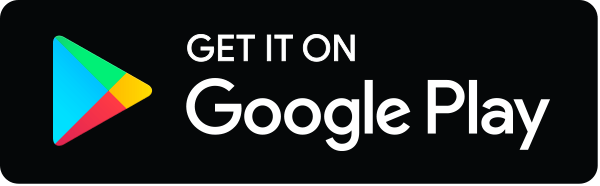