Objectives
The reader knows the structure, function, distribution, and control of the blood supply of the lung.
- Compares and contrasts the bronchial circulation and the pulmonary circulation.
- Describes the anatomy of the pulmonary circulation, and explains its physiologic consequences.
- Compares and contrasts the pulmonary circulation and the systemic circulation.
- Describes and explains the effects of lung volume on pulmonary vascular resistance.
- Describes and explains the effects of elevated intravascular pressures on pulmonary vascular resistance.
- Lists the neural and humoral factors that influence pulmonary vascular resistance.
- Describes the effect of gravity on pulmonary blood flow.
- Describes the interrelationships of alveolar pressure, pulmonary arterial pressure, and pulmonary venous pressure, as well as their effects on the regional distribution of pulmonary blood flow.
- Predicts the effects of alterations in alveolar pressure, pulmonary arterial and venous pressure, and body position on the regional distribution of pulmonary blood flow.
- Describes hypoxic pulmonary vasoconstriction and discusses its role in localized and widespread alveolar hypoxia.
- Describes the causes and consequences of pulmonary edema.
Blood Flow to the Lung: Introduction
The lung receives blood flow via both the bronchial circulation and the pulmonary circulation. Bronchial blood flow constitutes a very small portion of the output of the left ventricle and supplies part of the tracheobronchial tree with systemic arterial blood. Pulmonary blood flow (PBF) constitutes the entire output of the right ventricle and supplies the lung with the mixed venous blood draining all the tissues of the body. It is this blood that undergoes gas exchange with the alveolar air in the pulmonary capillaries. Because the right and left ventricles are arranged in series in normal adults, PBF is approximately equal to 100% of the output of the left ventricle. That is, PBF is equal to the cardiac output—normally about 3.5 L/min/m2 of body surface area at rest.
There is about 250 to 300 mL of blood per square meter of body surface area in the pulmonary circulation. About 60 to 70 mL/m2 of this blood is located in the pulmonary capillaries. It takes a red blood cell about 4 to 5 seconds to travel through the pulmonary circulation at resting cardiac outputs; about 0.75 of a second of this time is spent in pulmonary capillaries. Pulmonary capillaries have average diameters of around 6 μm; that is, they are slightly smaller than the average erythrocyte, which has a diameter of about 8 μm. Erythrocytes must therefore change their shape slightly as they pass through the pulmonary capillaries. An erythrocyte passes through a number of pulmonary capillaries as it travels through the lung. Gas exchange starts to take place in smaller pulmonary arterial vessels, which are not truly capillaries by histologic standards. These arterial segments and successive capillaries may be thought of as functional pulmonary capillaries. In most cases in this book, pulmonary capillaries refer to functional pulmonary capillaries rather than to anatomic capillaries.
About 280 billion pulmonary capillaries supply approximately 300 million alveoli, resulting in a potential surface area for gas exchange estimated to be 50 to 100 m2. As shown in Figure 1–4, the alveoli are completely enveloped in pulmonary capillaries. The capillaries are so close to one another that some researchers have described pulmonary capillary blood flow as resembling blood flowing through 2 parallel sheets of endothelium held together by occasional connective tissue supports.
The Bronchial Circulation
The bronchial arteries arise variably, either directly from the aorta or from the intercostal arteries. They supply arterial blood to the tracheobronchial tree and to other structures of the lung down to the level of the terminal bronchioles. They also provide blood flow to the hilar lymph nodes, visceral pleura, pulmonary arteries and veins, vagus, and esophagus. Lung structures distal to the terminal bronchioles, including the respiratory bronchioles, alveolar ducts, alveolar sacs, and alveoli, receive oxygen directly by diffusion from the alveolar air and receive nutrients from the mixed venous blood in the pulmonary circulation. The bronchial circulation may be important in the “air-conditioning” of inspired air, which is discussed in Chapter 10.
The blood flow in the bronchial circulation constitutes about 2% of the output of the left ventricle. Blood pressure in the bronchial arteries is the same as that in the other systemic arteries (disregarding differences due to hydrostatic effects, which will be discussed later in this chapter). This is much greater than the blood pressure in the pulmonary arteries (Figure 4–1). The reasons for this difference will be discussed in the next section.
The venous drainage of the bronchial circulation is unusual. Although some of the bronchial venous blood enters the azygos and hemiazygos veins, a substantial portion of bronchial venous blood enters the pulmonary veins. The blood in the pulmonary veins has undergone gas exchange with the alveolar air—that is, the pulmonary veins contain “arterial” blood. Therefore, the bronchial venous blood entering the pulmonary venous blood is part of the normal anatomic right-to-left shunt, which will be discussed in Chapter 5. Histologists have also identified anastomoses, or connections, between some bronchial capillaries and pulmonary capillaries and between bronchial arteries and branches of the pulmonary artery. These connections probably play little role in a healthy person but may open in pathologic states, such as when either bronchial or PBF to a portion of lung is occluded. For example, if PBF to an area of the lung is blocked by a pulmonary embolus, bronchial blood flow to that area increases. Bronchial blood flow increases after lung injury, and in inflammatory and proliferative diseases. The bronchial circulation is also the primary source of new vessels for the lung after injury. The main anatomic features of the bronchial circulation are illustrated in Figure 4–2.
Figure 4–2.
Illustration of the main anatomic features of the bronchial circulation. Note that the bronchial circulation supplies blood flow to the tracheobronchial tree down to the level of the terminal bronchioles as well as to the pulmonary blood vessels, the visceral pleura, the hilar lymph nodes, and branches of nerves, including the vagus. A bronchopulmonary anastomosis is shown at right and is enlarged in the inset. Venous drainage is to both the right side of the circulation via the azygos (and hemiazygos) vein and the left side of the circulation via the pulmonary veins. (Reproduced with permission from Deffebach, Charan, Lakshminarayan, and Butler, 1987.)
The Functional Anatomy of the Pulmonary Circulation
The pulmonary artery and its branches are much thinner-walled than corresponding parts of the systemic circulation. The pulmonary artery rapidly subdivides into terminal branches that have thinner walls and greater internal diameters than do corresponding branches of the systemic arterial tree. There is much less vascular smooth muscle in the walls of the vessels of the pulmonary arterial tree, and there are no highly muscular vessels that correspond to the systemic arterioles. The pulmonary arterial tree rapidly subdivides over a short distance, ultimately branching into the approximately 280 billion pulmonary capillaries, where gas exchange occurs.
The thin walls and small amount of smooth muscle found in the pulmonary arterial tree have important physiologic consequences. The pulmonary vessels offer much less resistance to blood flow than do the systemic arterial tree. They are also much more distensible than systemic arterial vessels. These factors lead to much lower intravascular pressures than those found in the systemic arteries, which makes them more compressible. The pulmonary vessels are located in the thorax and are subject to alveolar and intrapleural pressures that can change greatly.
Therefore, factors other than the tone of the pulmonary vascular smooth muscle may have profound effects on pulmonary vascular resistance (PVR). The transmural pressure difference across vessel walls is therefore a major determinant of PVR.
PVR cannot be measured directly but must be calculated. Poiseuille’s law, which states that for a Newtonian fluid flowing steadily through a nondistensible tube, is usually used to estimate PVR. This can be rearranged to:
For the pulmonary circulation, then,
That is, the PVR is equal to the mean pulmonary artery pressure (MPAP) minus the mean left atrial pressure (MLAP), with the result divided by PBF, which is equal to the cardiac output.
This formula, however, is only an approximation because blood is not a Newtonian fluid, because PBF is pulsatile (and may also be turbulent), because the pulmonary circulation is distensible (and compressible), and because the pulmonary circulation is a very complex branching structure. (Remember that resistances in series add directly; resistances in parallel add as reciprocals.) Furthermore, the MLAP may not be the effective downstream pressure for the calculation of PVR under all lung conditions (see the section on zones of the lung later in this chapter).
The intravascular pressures in the pulmonary circulation are lower than those in the systemic circulation (Figure 4–1). This is particularly striking with respect to the arterial pressures of the 2 circuits.
Because the right and left circulations are in series, the outputs of the right and left ventricles must be approximately equal to each other over the long run. (If they are not, blood and fluid will build up in the lungs or periphery.) If the 2 outputs are the same and the measured pressure drops across the systemic circulation and the pulmonary circulation are about 98 and 10 mm Hg, respectively, then the PVR must be about one tenth that of the systemic vascular resistance (SVR) (see Figure 4–1). (SVR is sometimes called TPR for total peripheral resistance.) This low resistance to blood flow offered by the pulmonary circulation is due to the structural aspects of the pulmonary circulation already discussed. The pulmonary vasculature is thinner walled, has much less vascular smooth muscle, and is generally more distensible than the systemic circulation.
The distribution of PVR can be seen by looking at the pressure drop across each of the 3 major components of the pulmonary vasculature: the pulmonary arteries, the pulmonary capillaries, and the pulmonary veins. In Figure 4–1, the resistance is fairly evenly distributed among the 3 components. At rest, about one third of the resistance to blood flow is located in the pulmonary arteries, about one third is located in the pulmonary capillaries, and about one third is located in the pulmonary veins. This is in contrast to the systemic circulation, in which about 70% of the resistance to blood flow is located in the systemic arteries, mostly in the highly muscular systemic arterioles.
The pressure at the bottom of a column of a liquid is proportional to the height of the column times the density of the liquid times gravity. Thus, when the normal mean systemic arterial blood pressure is stated to be about 100 mm Hg, the pressure developed in the aorta is equivalent to the pressure at the bottom of a column of mercury that is 100 mm high (it will push a column of mercury up 100 mm). Mercury is chosen for pressure measurement when high pressures are expected because it is a very dense liquid. Water is used when lower pressures are to be measured because mercury is 13.6 times as dense as water. Therefore, lower pressures, such as alveolar and pleural pressures, are expressed in centimeters of water.
Nevertheless, when mean arterial blood pressure is stated to be 100 mm Hg, this is specifically with reference to the level of the left atrium. Blood pressure in the feet of a person who is standing is much higher than 100 mm Hg because of the additional pressure exerted by the “column” of blood from the heart to the feet. In fact, blood pressure in the feet of a standing person of average height with a mean arterial blood pressure of 100 mm Hg is likely to be about 180 mm Hg. Because venous pressure is similarly increased in the feet (about 80 mm Hg), the pressure difference between arteries and veins is unaffected. Conversely, pressure decreases with distance above the heart (“above” with respect to gravity), so that blood pressure at the top of the head may only be 40 to 50 mm Hg.
The left ventricle, then, must maintain a relatively high mean arterial pressure because such high pressures are necessary to overcome hydrostatic forces and pump blood “uphill” to the brain. The apices of the lungs are a much shorter distance above the right ventricle, and so such high pressures are unnecessary.
A second consequence of the high arterial pressure in the systemic circulation is that it allows the redistribution of left ventricular output and the control of blood flow to different tissues. Because the left ventricle supplies all the tissues of the body with blood, it must be able to meet varying demands for blood flow in different tissues under various circumstances. For example, during exercise the blood vessels supplying the exercising muscle dilate in response to the increased local metabolic demand (and blood flow to the skin also increases to aid in thermoregulation). Blood pressure is partly maintained by increasing the resistance to blood flow in other vascular beds. A high-pressure head is thus necessary to allow such redistributions by altering the vascular resistance to blood flow in different organs; also, these redistributions help maintain the pressure head. In the pulmonary circulation, redistributions of right ventricular output are usually unnecessary because all alveolar-capillary units that are participating in gas exchange are performing the same function. The pressure head is low and the small amount of smooth muscle in the pulmonary vessels (which is in large part responsible for the low pressure head) makes such local redistributions unlikely. An exception to this will be seen in the section on hypoxic pulmonary vasoconstriction.
A final consequence of the pressure difference between the systemic and pulmonary circulations is that the workload of the left ventricle (stroke work equals stroke volume times arterial pressure) is much greater than that of the right ventricle. The metabolic demand of the left ventricle is also much greater than that of the right ventricle. The difference in wall thickness of the left and right ventricles of the adult is a reminder of the much greater workload of the left ventricle.
Pulmonary Vascular Resistance
The relatively small amounts of vascular smooth muscle, low intravascular pressures, and high distensibility of the pulmonary circulation lead to a much greater importance of extravascular effects (“passive factors”) on PVR. Gravity, body position, lung volume, alveolar and intrapleural pressures, intravascular pressures, and right ventricular output all can have profound effects on PVR without any alteration in the tone of the pulmonary vascular smooth muscle.
For distensible-compressible vessels, the transmural pressure difference is an important determinant of the vessel diameter. As the transmural pressure difference (which is equal to pressure inside minus pressure outside) increases, the vessel diameter increases and resistance falls; as the transmural pressure difference decreases, the vessel diameter decreases and the resistance increases. Negative transmural pressure differences lead to compression or even collapse of the vessel.
Two different groups of pulmonary vessels must be considered when the effects of changes in lung volume on PVR are analyzed: those vessels that are exposed to the mechanical influences of the alveoli and the larger vessels that are not—the alveolar and extraalveolar vessels (Figure 4–3).
Figure 4–3.
Illustration of alveolar and extraalveolar pulmonary vessels during an inspiration. The alveolar vessels (pulmonary capillaries) are exposed to the expanding alveoli and elongated. The extraalveolar vessels, here shown exposed to the intrapleural pressure, expand as the intrapleural pressure becomes more negative and as radial traction increases during the inspiration.
As lung volume increases during a normal negative-pressure inspiration, the alveoli increase in volume. While the alveoli expand, the vessels found between them, mainly pulmonary capillaries, are elongated. As these vessels are stretched, their diameters decrease, just as stretching a rubber tube causes its diameter to narrow. Resistance to blood flow through the alveolar vessels increases as the alveoli expand because the alveolar vessels are longer (resistance is directly proportional to length) and because their radii are smaller (resistance is inversely proportional to radius to the fourth power). At high lung volumes, then, the resistance to blood flow offered by the alveolar vessels increases greatly; at low lung volumes, the resistance to blood flow offered by the alveolar vessels decreases. This can be seen in the “alveolar” curve in Figure 4–4.
Figure 4–4.
The effects of lung volume on pulmonary vascular resistance (PVR). PVR is lowest near the functional residual capacity (FRC) and increases at both high and low lung volumes because of the combined effects on the alveolar and extraalveolar vessels. To achieve low lung volumes, one must generate positive intrapleural pressures so that the extraalveolar vessels are compressed, as seen at left in the figure. RV = residual volume; TLC = total lung capacity. (Reproduced with permission from Graph after Murray, 1976, 1986.)
One group of the extraalveolar vessels, the larger arteries and veins, is exposed to the intrapleural pressure. As lung volume is increased by making the intrapleural pressure more negative, the transmural pressure difference of the larger arteries and veins increases and they distend. Another factor tending to decrease the resistance to blood flow offered by the extraalveolar vessels at higher lung volumes is radial traction by the connective tissue and alveolar septa holding the larger vessels in place in the lung. (Look at the small branch of the pulmonary artery at the bottom of Figure 1–3.) Thus, at high lung volumes (attained by normal negative-pressure breathing), the resistance to blood flow offered by the extraalveolar vessels decreases (Figure 4–4). During a forced expiration to low lung volumes, however, intrapleural pressure becomes very positive. Extraalveolar vessels are compressed, and as the alveoli decrease in size, they exert less radial traction on the extraalveolar vessels. The resistance to blood flow offered by the extraalveolar vessels increases greatly (see left side of Figure 4–4).
Because the alveolar and extraalveolar vessels may be thought of as 2 groups of resistances in series with each other, the resistances of the alveolar and extraalveolar vessels are additive at any lung volume. Thus, the effect of changes in lung volume on the total PVR gives the U-shaped curve seen in Figure 4–4. PVR is lowest near the functional residual capacity and increases at both high and low lung volumes.
A second type of extraalveolar vessel is the so-called corner vessel, or extraalveolar capillary (see the circled asterisk in Figure 1–3). Although these vessels are found between alveoli, their locations at junctions of alveolar septa give them different mechanical properties. Expansion of the alveoli during inspiration increases the wall tension of the alveolar septa, and the corner vessels are distended by increased radial traction, whereas the alveolar capillaries are compressed.
Also note that during mechanical positive-pressure ventilation, alveolar pressure (PA) and intrapleural pressure are positive during inspiration. In this case, both the alveolar and extraalveolar vessels are compressed as lung volume increases, and the resistance to blood flow offered by both alveolar and extraalveolar vessels increases during lung inflation. This is especially a problem during mechanical positive-pressure ventilation with positive end-expiratory pressure (PEEP). During PEEP, airway pressure (and thus alveolar pressure) is kept positive at end expiration to help prevent atelectasis. In this situation, alveolar pressure and intrapleural pressure are positive during both inspiration and expiration. PVR is elevated in both alveolar and extraalveolar vessels throughout the respiratory cycle. In addition, because intrapleural pressure is always positive, the other intrathoracic blood vessels are subjected to decreased transmural pressure differences; the venae cavae, which have low intravascular pressure, are also compressed. If cardiovascular reflexes are unable to adjust to this situation, cardiac output may fall precipitously because of decreased venous return and high PVR.
During exercise, cardiac output can increase several-fold without a correspondingly great increase in MPAP. Although the MPAP does increase, the increase is only a few millimeters of mercury, even if cardiac output has doubled or tripled. Since the pressure drop across the pulmonary circulation is proportional to the cardiac output times the PVR (ie, ), this must indicate a decrease in PVR.
Like the effects of lung volume on PVR, this decrease appears to be passive—that is, it is not a result of changes in the tone of pulmonary vascular smooth muscle caused by neural mechanisms or humoral agents. In fact, a fall in PVR in response to increased blood flow or even an increase in perfusion pressure can be demonstrated in a vascularly isolated perfused lung, as was used to obtain the data summarized in Figure 4–5.
In this experiment, the blood vessels of the left lung of a dog were isolated, cannulated, and perfused with a pump. The lung was ventilated with a mechanical respirator. Blood flow to the lung and MPAP were elevated by increasing the pump output. As can be seen from the graph, increasing the blood flow to the lung caused a decrease in the calculated PVR. Increasing the left atrial pressure also decreased PVR in these studies.
There are 2 different mechanisms that can explain this decrease in PVR in response to elevated blood flow and perfusion pressure: recruitment and distention (Figure 4–6).
Figure 4–6.
Illustration of the mechanisms by which increased mean pulmonary artery pressure may decrease pulmonary vascular resistance. The upper figure shows a group of pulmonary capillaries, some of which are perfused. At left, the previously unperfused capillaries are recruited (opened) by the increased perfusion pressure. At right, the increased perfusion pressure has distended those vessels already open.
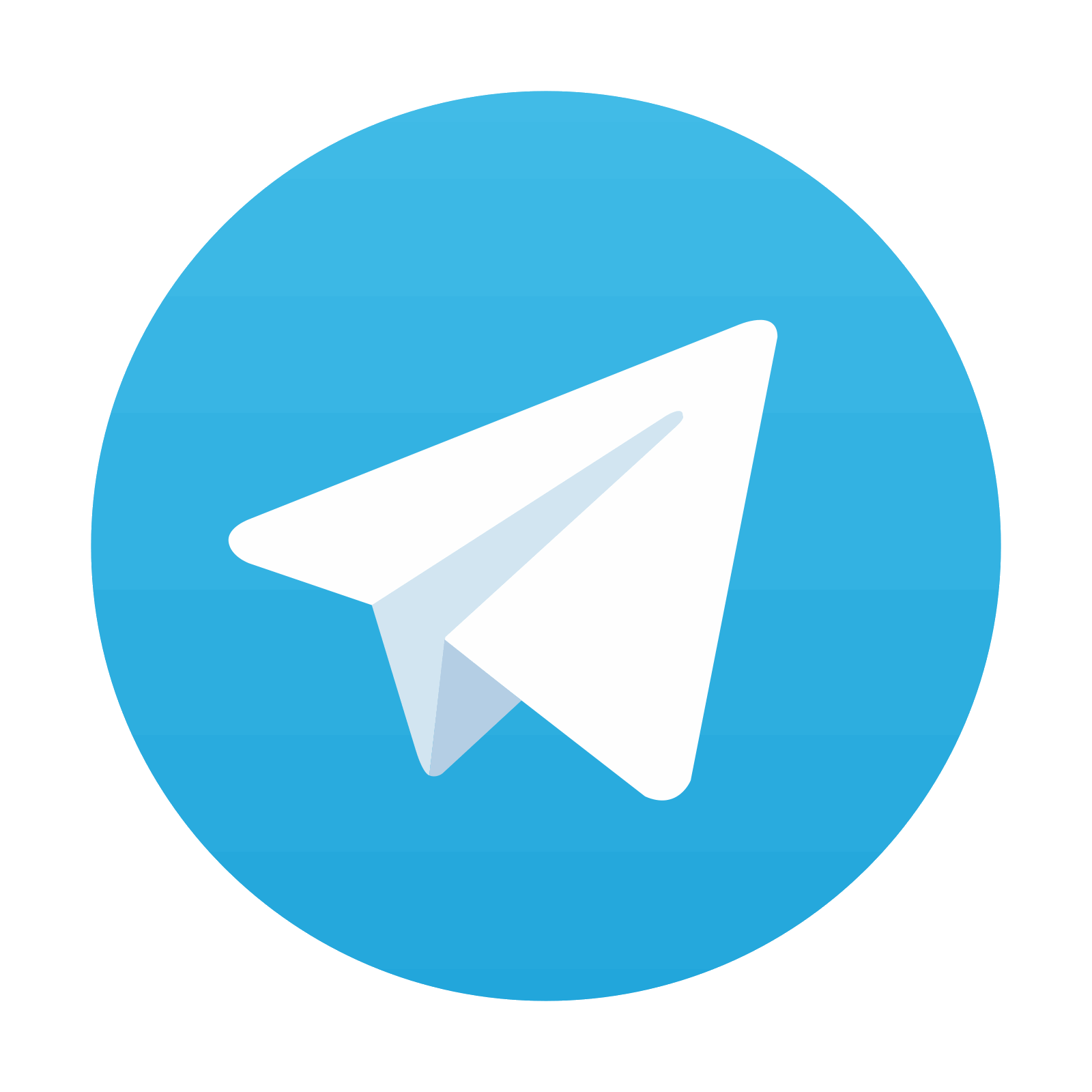
Stay updated, free articles. Join our Telegram channel
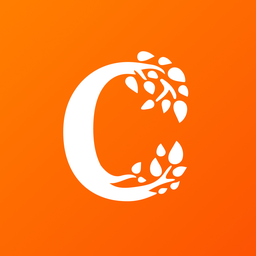
Full access? Get Clinical Tree
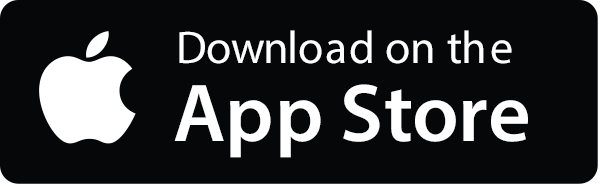
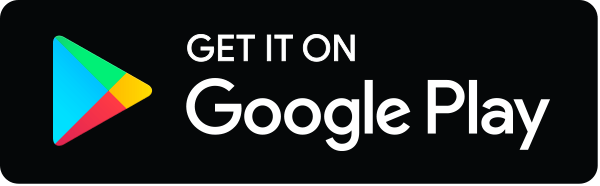