Chapter 27 The general term lipid applies to a class of hydrophobic compounds that are soluble in organic solvents and nearly insoluble in water. Chemically, lipids are usually enriched in carbon and hydrogen and after hydrolysis typically yield fatty acids or complex alcohols, which are usually esterified with fatty acids. Some lipids are more complex, containing other chemical groups, such as sialic, phosphoryl, amino, or sulfate groups. The presence of these charged or polar groups makes these lipids amphipathic, which gives them the property of having an affinity for both water and organic solvents; this is an important feature in their ability to form cell membranes. Lipids can been broadly subdivided into five groups based on their chemical structure (Box 27-1). Although every living organism has been found to contain sterols, cholesterol is found almost exclusively in animals, in which it is also the main sterol. Virtually all cells and body fluids contain some cholesterol. Similar to other sterols, cholesterol is a solid alcohol of high molecular weight that possesses a tetracyclic perhydrocyclopentanophenanthrene skeleton. It contains 27 carbon atoms, numbered as shown in Figure 27-1. Knowledge of the sterane skeleton structure and numbering system is important not only to clinical laboratorians but also to practicing clinicians, because cholesterol is the starting point in many different metabolic pathways. These include vitamin D synthesis (see Chapter 52), steroid hormone synthesis (see Chapter 53), and bile acid metabolism (see Chapter 50). Because the enzymes modifying the sterane cholesterol ring or its derivatives are known by their site and type of reaction (e.g., 21-hydroxylase in cortisol synthesis), the diagnosis of many disease states consequently depends on isolating the site of enzyme dysfunction (e.g., 21-hydroxylase deficiency in adrenogenital syndrome). After its absorption into the intestinal mucosal cell, cholesterol, together with triglycerides, phospholipids, and a number of specific apoproteins, is assembled into a large lipoprotein called a chylomicron (see later section on lipoprotein metabolism, exogenous pathway). One apoprotein component known as apolipoprotein (apo) B-48 is vital in the formation and secretion of chylomicrons. Patients with a rare deficiency in this process develop a disease called chylomicron retention disorder, which is characterized by excess lipid in enterocytes and fat malabsorption. Once secreted by enterocytes, chylomicrons enter the lymphatics, which eventually empty into the thoracic duct and enter the systemic venous circulation at the junction of the left subclavian vein and the left internal jugular vein. Although cholesterol enters the body from the diet, it is also synthesized endogenously by all tissues from acetate. Knowledge of the endogenous cholesterol synthetic pathway has assumed great significance, because drug agents for the treatment of CHD have been sought to suppress or decrease endogenous cholesterol synthesis. The necessity for understanding the fundamental biochemistry of this pathway was originally underscored by the triparanol disaster of 1960. Triparanol is a drug that inhibits the final step in the endogenous cholesterol synthetic pathway—the conversion of desmosterol to cholesterol—but does not inhibit the rate-limiting enzyme of cholesterol synthesis, 3-hydroxy-3-methylglutaryl-CoA (HMG-CoA) reductase (Figure 27-2). When triparanol was used to treat hypercholesterolemia, the drug caused tissue accumulation of desmosterol, resulting in the development of cataracts, alopecia, and accelerated atherosclerosis. Many drugs now used to treat CHD selectively suppress the rate-limiting enzyme HMG-CoA reductase, thereby lowering serum cholesterol concentrations significantly without accumulation of water-insoluble intermediates of cholesterol synthesis, such as desmosterol. Although essentially all cells have the capacity to synthesize cholesterol from acetyl-CoA, almost 90% of synthesis occurs in the liver and gut; other organs and tissues depend, in part, on cholesterol delivery from the circulation. Cholesterol biosynthesis is best conceptualized as occurring in three stages (Figures 27-2 through 27-4). In the first stage, acetyl-CoA, a key metabolic intermediate that can be derived from carbohydrates, amino acids, and fatty acids, forms the six-carbon thioester HMG-CoA. In the second stage, HMG-CoA is reduced to mevalonate, then is decarboxylated to form five-carbon isoprene units. These isoprene units are condensed to form first a 10-carbon (geranyl pyrophosphate) and then a 15-carbon intermediate (farnesyl pyrophosphate). Two of these C15 molecules combine to produce the final product of the second stage—squalene, a 30-carbon acyclic hydrocarbon. The second stage is important, because it contains the step involving the microsomal enzyme HMG-CoA reductase, which is the rate-limiting enzyme in cholesterol biosynthesis. The enzyme that forms farnesyl pyrophosphate, geranyl transferase, is an important second site of regulation in cholesterol synthesis. This degree of inhibition still permits the formation of physiologically important intermediate isoprenoids in the absence of cholesterol synthesis. The third and final stage of synthesis occurs in the endoplasmic reticulum, with many of the intermediate products being bound to a specific carrier protein. Squalene, after initial oxidation, undergoes cyclization to form the four-ring, 30-carbon intermediate, lanosterol. In a series of oxidation-decarboxylation reactions, several sidechains are removed from the pentanophenanthrene structure to form the final 27-carbon molecule of cholesterol. Once synthesized, cholesterol is released into the circulation bound to lipoproteins, such as very low-density lipoprotein (VLDL; see later section on lipoprotein metabolism, endogenous pathway), which is one of the primary lipoproteins secreted by the liver. The major apoprotein found in VLDL is apo B-100. It is produced by the same gene as apo B-48, but represents the full-length product of the apo B gene. Apo B-48 is produced in the intestine by a post-transcriptional editing step, which introduces a stop codon in about the middle of the apo B-100 mRNA transcript, thus resulting in a protein about 48% the length of the full-length apo B-100 protein. The esterification of cholesterol is important, because it serves to enhance the lipid-carrying capacity of the lipoprotein in plasma and prevents intracellular toxicity by free cholesterol. The reaction is catalyzed by lecithin-cholesterol acyltransferase (LCAT) in the plasma and acylcholesterol acyltransferase (ACAT) within the cell. ACAT is an energy-requiring enzyme, and the initial reaction (Figure 27-5) involves activation of a fatty acid with thio coenzyme A (Co-ASH) to form an acyl-CoA, which in turn reacts with cholesterol to form an ester. The LCAT reaction does not require CoASH and results from fatty acid transfer from the second carbon position of phosphatidyl choline (lecithin) to the hydroxyl group on the A-ring of cholesterol (see Figure 27-5). Cholesteryl esters account for about 70% of the total cholesterol in plasma, and LCAT is responsible for the formation of virtually all plasma cholesteryl ester. LCAT is synthesized in the liver and released into the circulation; it primarily resides on lipoproteins and is activated by apo A-I and other apolipoproteins (see later section on apolipoproteins). The esterification of cholesterol by LCAT on the only polar group of cholesterol makes cholesteryl ester more hydrophobic than cholesterol. This causes the cholesterol on the surface of lipoproteins, once esterified, to partition into the more hydrophobic core of lipoproteins, where triglycerides are also located. Cholesterol reaching the liver may be secreted unchanged into bile or metabolized to bile acids or resecreted on lipoproteins. Approximately one third of the daily production of cholesterol, or about 400 mg/d, is converted into bile acids (Figure 27-6). Conversion of cholesterol to cholic and chenodeoxycholic acids, the major bile acids in humans, involves shortening of the cholesterol sidechain and hydroxylation of the sterol nucleus. The first step, which is also the rate-limiting step, is hydroxylation of the 7-position of the sterol nucleus, catalyzed by the enzyme 7α-hydroxylase (see Figure 27-6). The bile acids are made more polar after conjugation with glycine or taurine and then are excreted into the bile canaliculi. After reaching the small intestine, the conjugated bile acids play an active part in cholesterol and fat absorption, as discussed previously. Some of the bile acids are deconjugated and converted by bacteria in the intestine to secondary bile acids. Cholic acid is converted to deoxycholic acid, and chenodeoxycholic acid is metabolized to lithocholic acid. About 90% of the bile acids, except lithocholic, are reabsorbed in the lower third of the ileum and returned to the liver by the portal vein, which is called the enterohepatic circulation (see also Chapter 50). Fatty acids are further classified according to their degree of saturation. Saturated fatty acids have no double bonds between carbon atoms, monounsaturated fatty acids contain one double bond, and polyunsaturated fatty acids contain more than one double bond (Figure 27-7). The double bonds in polyunsaturated fatty acids of both animal and plant origin are usually 3 carbon atoms apart. Some fatty acids from marine fish living in deep, cold waters (e.g., salmon), which form oils at room temperature, possess numerous (up to 6) unsaturated bonds and usually are more than 20 carbon atoms long. These fatty acids are prone to oxidation, which occurs at the sites of unsaturation. Labeling of carbon atoms in fatty acids can take place from the carboxyl terminal (Δ-numbering system) or from the methyl terminal (η- or ω-numbering system; Table 27-1). In addition, carbon atoms may be labeled with Greek symbols, with α being adjacent to the carboxyl group and ω being farthest away. In the Δ-system, fatty acids are abbreviated according to the number of carbon atoms, the number of double bonds, and the position(s) of double bond(s). For example, linoleic acid, which contains 18 carbons and 2 unsaturated bonds between carbons 9 and 10 and between carbons 12 and 13, could be written as C18:2.9,12 Using the η- or ω-system, linoleic acid would be abbreviated to C18:2n-6, where only the first carbon forming the unsaturated pair is written. The Geneva or systematic classification is a third system of nomenclature (see Table 27-1). In saturated fatty acids, the acyl chain is extended and flexible (i.e., the carbon atoms rotate freely around the longitudinal axis), and each internal carbon atom is fully saturated or, in other words, is covalently linked to two hydrogen molecules. Cis-unsaturated fatty acids have a fixed 30° bend in their acyl chains at each double bond, because two hydrogen molecules are missing from the same side of the carbon double bond, thus causing the bend. Lipids containing cis-unsaturated fatty acids, such as triglycerides or phospholipids, have lower melting points, because these lipids cannot interact and pack as tightly together by van der Waals interactions. As a consequence, lipids containing cis-unsaturated fatty acids, such as olive oil and other plant oils, are usually liquids at room temperature. In mammals, all naturally occurring unsaturated fatty acids are of the cis variety. Trans unsaturated fatty acids result from a chemical process called catalytic hydrogenation, a process used to “harden” unsaturated fats from plant sources in the manufacture of certain foods, such as margarine. Although trans fatty acids are still unsaturated, one hydrogen is missing from each side of the carbon double bond, making these fatty acids resemble the more linear configuration of the acyl chain of saturated fatty acids, which also makes them solids at room temperature. Epidemiologic and experimental studies have shown that trans fatty acids may promote CHD; an effort is under way to reduce the use of catalytic hydrogenation in food processing and to reduce the consumption of trans fatty acids in the diet.203 Most fats in the human body are derived from the diet, which on the average in Western societies contains up to 40% fat, 90% of which is triglyceride. In addition, humans can synthesize most fatty acids, including saturated, monounsaturated, and some polyunsaturated fats; however, some fatty acids cannot be synthesized. One such fatty acid is linoleic acid (C18:29,12), which is found only in plants. Because it is vital for health and growth and development, it is termed an essential fatty acid. Linoleic acid is converted to arachidonic acid, which has an important role in prostaglandin synthesis and in myelinization of the central nervous system. Long-chain fatty acids are oxidized in the mitochondria and produce energy by a series of reactions that operate in a repetitive manner to shorten the fatty acid chain by two carbon atoms at a time from the carboxy (—COOH) terminal of the molecule through a process known as β-oxidation. For example, 1 mole of C16 fatty acid is converted to 8 moles of acetyl-CoA. Acetyl-CoA does not normally accumulate in the cell but is enzymatically condensed with oxaloacetate, derived largely from carbohydrate metabolism (Figure 27-8), to yield citrate, which is a major component of the tricarboxylic acid cycle, also called Krebs cycle. The Krebs cycle serves as a common pathway for the final oxidation of nearly all food material, whether derived from carbohydrate, fat, or protein. It is important to note that the efficiency of the Krebs cycle depends on the availability of sufficient oxaloacetate to serve as an acceptor for acetyl-CoA. Complete oxidation of a single fatty acid molecule produces a relatively large quantity of energy. For example, the complete oxidation of 1 mole of palmitic acid to carbon dioxide and water produces 16 moles of CO2, 16 moles of H2O, and 129 moles of adenosine triphosphate, or 2340 Cal.* Thus the standard free energy for oxidation of palmitic acid is 2340 Cal, whereas the free energy liberated by hydrolysis of 129 moles of ATP is 940 Cal, indicating that the efficiency of energy conservation in fatty acid oxidation is approximately 40% under standard conditions. During prolonged starvation, or when carbohydrate metabolism is severely impaired, as in uncontrolled diabetes mellitus (see Chapter 26), the formation of acetyl-CoA exceeds the supply of oxaloacetate. The abundance of acetyl-CoA results from excessive mobilization of fatty acids from adipose tissue and excessive degradation of fatty acids by β-oxidation in the liver. The resulting acetyl-CoA excess is diverted to an alternative pathway in the mitochondria and forms acetoacetic acid, β-hydroxybutyric acid, and acetone—three compounds known collectively as ketone bodies (Figure 27-9). The presence of ketone bodies is a frequent finding in severe, uncontrolled diabetes mellitus. As shown in Figure 27-9, the first product, acetoacetyl-CoA, condenses in the mitochondria with a third molecule of acetyl-CoA to yield HMG-CoA. This pool of HMG-CoA in the mitochondria is distinct from the pool in the cytosol, which is used for cholesterol biosynthesis. The HMG-CoA produced in the mitochondria is cleaved enzymatically to yield acetoacetate and acetyl-CoA. Some of the acetoacetate formed in liver cells is usually reduced to β-hydroxybutyrate. Because acetoacetate is unstable, a further portion decomposes to form carbon dioxide and acetone, the third ketone body found in severe, untreated diabetes mellitus. Ketosis, therefore, develops from excessive production of acetyl-CoA because the body attempts to derive necessary energy from stored fat in the absence of an adequate supply of carbohydrate metabolites (see Chapter 26). The entire process of ketosis is reversed by restoring adequate metabolism of carbohydrate. In starvation, restoration consists of adequate carbohydrate ingestion; in diabetes mellitus, ketosis can be reversed by insulin administration, which permits circulating blood glucose to be taken up by the cells. With restored concentrations of oxaloacetate, acetyl-CoA can instead enter the Krebs cycle, thus restoring the normal pathway for energy metabolism. Eventually, the release of fatty acids from adipose tissue slows down and is finally reversed. A graphic view of these metabolic reactions is outlined in Figure 27-8, which shows the interrelationship between carbohydrate, fatty acid, and protein metabolism. By convention, prostaglandins are abbreviated PG, with the class designated by a capital letter (A, B, E, F, G, H, and I), followed by a number and then in some cases a Greek letter (Figure 27-10). With the exception of PGG and PGH, which have the same ring structure (cyclopentane endoperoxide), the letters refer to different ring structures. PGA and PGB have keto groups at C-9, with the A series having a double bond between C-10 and C-11, and the B series having a double bond between C-8 and C-12. PGE also has a C-9 keto bond but a hydroxyl group at C-11. The F series has hydroxyl groups at both C-9 and C-11. The difference between PGG and PGH, which have identical ring structures, occurs in the sidechain at C-15 in R2. The G series has a peroxide group, whereas the H series has a hydroxyl group. The I series has a double-ring formation, with the C-9 of the cyclopentane ring linked to C-6 of the sidechain by an oxygen molecule to form a second five-sided ring (see Figure 27-10). The endoperoxide PGs (G and H series) are intermediates in the formation of other PGs. The number after the capital letter is usually written as a subscript and is used to designate the number of unsaturated bonds in the PG sidechains and not within the ring structure itself. In PGE1, for example, a double bond exists between C-13 and C-14; in the 2 series (PGE2), a double bond exists between C-13 and C-14 and between C-5 and C-6; and in the 3 series (PGE3), an additional double bond occurs between C-17 and C-18. The 2 series is most common. The bond between C-13 and C-14 is always trans, whereas bonds between C-5 and C-6 and between C-17 and C-18 are always cis. At C-15, all naturally occurring prostaglandins have a hydroxyl group that projects below the plane of the ring. Use of the Greek letter (α or β) applies only to the F series and refers to the hydroxyl group found at C-9. In the α-series, the hydroxyl group projects below the ring plane in the same direction as the C-11 hydroxyl group, whereas the β-series denotes that the hydroxyl at C-9 is above the plane of the ring. Sixteen naturally occurring prostaglandins have been described (Table 27-2), but only seven, along with two thromboxanes, are commonly found throughout the body, and they are termed the primary prostaglandins. TABLE 27-2 Naturally Occurring Prostaglandins (PGs) Although prostaglandins have hormone-like actions, they are different from most other hormones in that (1) they are synthesized at the site of action, and (2) they are made in almost all tissues. Linoleic acid (C18:29,12) is the precursor of two of the three 20-carbon fatty acids that form prostaglandins; linolenic acid (C18:29,12,15) is the other precursor. Both of these fatty acids are considered essential because they cannot be synthesized in the body and, therefore, must be present in the diet. The three C20 fatty acids subsequently formed are C20:35,8,11 (eicosatrienoic acid), C20:45,8,11,14 (eicosatetraenoic or arachidonic acid), and C20:58,11,14,17(eicosatrienoic acid). These three fatty acids form the PG1, PG2, and PG3 series, respectively. Once formed, prostaglandins exert very short-lived effects and are rapidly catabolized to inactive forms within a few seconds. Inactivation of prostaglandin appears to be mediated by two enzymes: 15 α-hydroxy-prostaglandin dehydrogenase and Δ13-prostaglandin reductase. Prostaglandins are not stored; instead, precursor C20 fatty acids are present in tissue attached to the C-2 (see later section in this chapter on glycerol esters) of phosphoglycerides. When necessary, the C20 precursor is hydrolyzed by phospholipase A2, which is specific for the C-2 position of phosphoglyceride. Release of the C20 fatty acid appears to be the rate-limiting step in prostaglandin synthesis and is stimulated by many different agents, such as bradykinin, thrombin, or angiotensin II. Although it is probable that all prostaglandins follow a similar synthetic pathway, C20:4 (arachidonic acid) has been the most intensively studied and is used to illustrate the pathway (Figure 27-11). Once released, arachidonic acid follows one of two pathways. The lipoxygenase route produces 12-L-hydroperoxy-5,8,10,14 eicosatetraenoic acid (HPETE); HPETE then spontaneously decomposes to 12-L-hydroxy-5,8,10,14 eicosatetraenoic acid (HETE). The alternative pathway is mediated by cyclooxygenase (COX) to produce the endoperoxides PGG2 and PGH2. The latter can be degraded to 12-L-hydroxy-5,8,10-heptadecatrienoic acid. What controls the entry into a specific pathway remains speculative; however, it is known that nonsteroidal anti-inflammatory drugs (NSAIDs; aspirin, ibuprofen, and indomethacin) inhibit the COXs, thereby decreasing prostaglandin synthesis. Two isoforms of COX are known: COX-1 and COX-2. COX-1 concentrations in general are constitutively expressed, whereas COX-2 is synthesized in response to inflammation. Certain drugs that inhibit both COXs have nephrotoxic and ulcerogenic side effects. Therefore, newer NSAIDs have been developed that preferentially inhibit COX-2 to reduce side effects, but they have been found to also be associated with an increased incidence of myocardial infarction.94 Prostaglandin I2, or prostacyclin, is derived from arachidonic acid (see Figure 27-11) in the vascular endothelium. It has a powerful vasodilatory action, especially on the coronary arteries, and is responsible for inhibiting platelet aggregation. Thromboxane A2 is synthesized from arachidonic acid but is also produced by platelets. It has the opposite effect of prostacyclin (i.e., it stimulates the contraction of arterial smooth muscle and enhances platelet aggregation). It has a very short half-life—about 30 seconds—and is rapidly converted to its inactive metabolite, thromboxane B2. The thromboxanes are slightly different from other prostaglandins in that they contain six-sided rings of five carbon atoms and one oxygen atom (Figure 27-12). Table 27-3 lists some reported functions of the various prostaglandins. TABLE 27-3 Prostaglandin-Mediated Effects The class of acylglycerol (glyceride) is determined by the number of fatty acyl groups present; one fatty acid, monoacylglycerols (monoglycerides); two fatty acids, diacylglycerols (diglycerides); three fatty acids, triacylglycerols (triglycerides). In a monoacylglycerol, the fatty acid may be linked to any of the three carbon atoms. By convention, the number system is used to indicate the carbon position (e.g., 1-monoglyceride indicates a fatty acid attachment to the α-carbon). This numbering system applies to all acylglycerols, including the phosphoglycerides, as shown later. Diglycerides may be 1,2- or 1,3-diglycerides (Figure 27-13). Triglycerides are the most prevalent glycerol esters encountered in the body and constitute 95% of tissue storage fat; they are the predominant form of glyceryl ester found in plasma. Fatty acid residues found in monoglycerides, diglycerides, or triglycerides vary considerably and usually include combinations of the long-chain fatty acids shown in Table 27-1. Triglycerides from plants (e.g., corn, sunflower seed, safflower oils) tend to have large quantities of cis-unsaturated fatty acids, such as C18:2 or linoleic acid, and are liquid at room temperature. Triglycerides from animals, especially ruminants, tend to have C12:0 through C18:0 fatty acid residues (saturated fats) and are solids at room temperature. Rarely, some plant triglycerides, such as coconut oil, are highly saturated and may be solid at room temperature. Triglycerides are digested in the duodenum and the proximal ileum. Through the action of pancreatic and intestinal lipases and in the presence of bile acids, which activate lipases, they are hydrolyzed to glycerol, monoglycerides, and fatty acids. After absorption, triglycerides are resynthesized in the intestinal epithelial cells and are combined with cholesterol and apo B-48 to form chylomicrons. Another major class of glycerol esters consists of those containing phosphoric acid at the third (α′) carbon atom; these esters are called phosphoglycerides (Figure 27-14). In their simplest form, the A group is an H atom; the molecule is therefore called a diacylphosphoglyceride. Usually, however, the A is an alcohol-derived group, such as choline, serine, inositol, or ethanolamine (see Figure 27-14). If A is choline, the molecule is referred to as phosphatidylcholine; if it is ethanolamine, it will be referred to as phosphatidylethanolamine, etc. The term lecithin, which is an older designation, is a commonly used term for phosphatidylcholines. Because of the wide variety of fatty acid residues at positions R1 and R2 (see Figure 27-14), many different types of phospholipids can be formed. These phospholipids are named according to the fatty acid acyl ester attached at C-1 and C-2 of the glycerol. Saturated fatty acids are typically attached to the C-1 position, whereas (poly)unsaturated fatty acids are often present at the C-2 position. In inner mitochondrial membranes, more complex phosphoglycerides known as cardiolipins can be found. They are derived from two phosphoglyceride molecules joined by a glycerol bridge. Sphingolipids, a fourth class of lipids found in humans, are derived from the amino alcohol sphingosine (Figure 27-15). This dihydric 18-carbon alcohol contains an amino group at C-17. A fatty acid containing 18 or more carbon atoms can be bound to the amino group through an amide linkage to form ceramide. This is the intermediary step in the formation of three important sphingolipids: sphingomyelin, galactosylceramide, and glucosylceramide (see Figure 27-15). The sugar-containing ceramides can have a sulfate group attached (usually on the 2-position of the galactose residue) to form the sulfatides. The glycosyl ceramides can have additional monosaccharide moieties, such as galactose, N-acetylgalactosamine, and N-acetylneuraminic acid to form complex globosides and gangliosides. These complex sphingolipids form the major lipids of cell membranes, particularly in the central nervous system. Gangliosides, for example, are particularly prevalent in the gray matter of the brain, whereas membrane glycosphingolipids have major roles in cellular interactions, growth, and development. Some glycolipids on red cells form blood group antigens, whereas other glycolipids can be tumor antigens. Lipids synthesized in the liver and the intestine must be transported to various tissues to accomplish their metabolic functions. Because of their relative insolubility in aqueous solutions, they are transported in the plasma in macromolecular complexes called lipoproteins. Lipoproteins are typically spherical particles with more hydrophobic nonpolar lipids (triglycerides and cholesterol esters) in their core, and more polar or amphipathic lipids (phospholipids and free cholesterol) oriented near the surface as a single monolayer. They also contain one or more specific proteins, called apolipoproteins, which usually are located on their surface (Figure 27-16).266 The association of core lipids with the overlying phospholipid, cholesterol, and protein coat is noncovalent, occurring primarily through hydrogen bonding and van der Waals forces. This binding is loose enough to allow spontaneous exchange of cholesterol, which is more water soluble than the other lipids, among plasma lipoproteins and between cell membranes. The other more hydrophobic lipids require specific transfer proteins to exchange between lipoproteins, such as cholesteryl ester transfer protein (CETP), which exchanges triglycerides and cholesteryl esters between lipoproteins. Phospholipid transfer protein (PLTP) promotes the transfer of phospholipids between lipoproteins. Lipoproteins have different physical and chemical properties (Table 27-4) because they contain different proportions of lipids and proteins (Table 27-5). Historically, lipoproteins have been categorized on the basis of differences in their hydrated densities, as determined by ultracentrifugation. Categories include (1) chylomicron, (2) VLDL, (3) intermediate-density lipoprotein (IDL), (4) low-density lipoprotein (LDL), (5) high-density lipoprotein (HDL), and (6) lipoprotein(a) [Lp(a)]. HDL can be further divided by density into two subpopulations: HDL2 and HDL3. Lp(a) consists of a distinct class of lipoproteins (see Table 27-4) that are structurally related to LDL, because similar to LDL, it contains one apo B-100 per particle and has a similar lipid composition.79,177 In contrast to LDL, Lp(a) contains a carbohydrate-rich protein called apo(a), which is covalently bound to apo B-100 through a disulfide linkage. Apo(a) exhibits a significant sequence homology with plasminogen, but unlike plasminogen, Lp(a) is not an active protease. Lp(a) contains a high degree of variation in polypeptide chain length because of a variable number of kringle domains (Figure 27-17). Apo(a) contains 10 distinct classes of kringle 4–like domains that differ from each other in amino acid sequence. Kringle 4 type 1 and kringle 4 types 3 to 10 are present as a single copy, but kringle 4 type 2 is present in variable numbers of repeats (3 to >40) (see Figure 27-17).157,323
Lipids, Lipoproteins, Apolipoproteins, and Other Cardiovascular Risk Factors
Basic Biochemistry
Basic Lipid Biochemistry
Cholesterol
Cholesterol Absorption
Cholesterol Synthesis
Cholesterol Esterification
Cholesterol Catabolism
Fatty Acids
Fatty Acid Catabolism
Ketone Formation
Prostaglandins
Primary PG
Other PG
PGE1
PGA1
PGF1α
PGA2
PGE2
19α-OHPGA1
PGF2α
19α-OHPGA2
PGG2
PGB1
PGH2
PGB2
PGI2
19α-OHPGB2
Thromboxane A2
PGE3
Thromboxane B2
PGF3α
Site of Action
Physiologic Response
Arterial smooth muscle
Alters blood pressure
Uterine muscle
Induces labor, therapeutic abortion
Lower gastrointestinal tract
Increases motility
Bronchial smooth muscle
Bronchospasm
Platelets
Increases coagulability
Capillaries
Increases permeability
Stomach
Enhances gastric acid secretion
Adipose tissue
Inhibits triglyceride lipolysis
Glycerol Esters (Acylglycerols)
Sphingolipids
Lipoproteins
Stay updated, free articles. Join our Telegram channel
Full access? Get Clinical Tree
Lipids, Lipoproteins, Apolipoproteins, and Other Cardiovascular Risk Factors
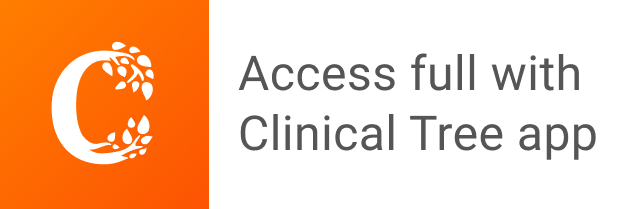