Chapter 48 Michael P. Delaney, B.Sc., M.D., F.R.C.P., Christopher P. Price, Ph.D., F.R.S.C., F.R.C.Path. and Edmund J. Lamb, Ph.D., F.R.C.Path.* The kidneys play a central role in the homeostatic mechanisms of the human body, and reduced renal function strongly correlates with increasing morbidity and mortality. Biochemical investigations, both routine and specialized, are an important part of the clinician’s diagnostic armamentarium, and investigations of kidney function constitute a significant element of the workload of most laboratories. The aim of this chapter is to ensure that the clinical chemist/biochemist understands the perspective of the nephrologist when dealing with laboratory investigations for patients with kidney disease. The basic anatomy and physiology of the kidneys are described as a foundation for understanding the pathophysiology of disease and the rationale for diagnostic and management strategies in kidney disease. Key analytical methods employed during the investigation of kidney disease are discussed in Chapter 25. The kidneys form a paired organ system located in the retroperitoneal space. They extend from the the lower part of the eleventh thoracic vertebra to the upper portion of the third lumbar vertebra, with the right kidney situated slightly lower than the left. The adult kidney is about 12 cm long and weighs about 150 g (Figure 48-1). The kidneys have both sympathetic and parasympathetic nerve supplies, whose function appears to be predominantly associated with vasomotor activity. The renal lymphatic drainage includes fine lymphatics in the glomerulus, some in close proximity to the juxtaglomerular apparatus (JGA; see Box 48-1 for a list of abbreviations), which are associated with removal of material from the glomerular mesangial cells. In most cases, each kidney receives its blood supply from a single renal artery derived from the abdominal aorta. However, multiple renal arteries occur commonly. The renal artery divides into posterior and anterior elements, and ultimately into the afferent arterioles, which expand into the highly specialized capillary beds that form the glomerulus (Figure 48-2). These capillaries then rejoin to form the efferent arteriole that forms the capillary plexuses and the elongated vessels (the vasa recta) that pass around the remaining parts of the nephron, the proximal and distal tubules, the loop of Henle, and the collecting duct, providing oxygen and nutrients and removing ions, molecules, and water, which have been reabsorbed by the nephron. The efferent arteriole then merges with renal venules to form the renal veins, which merge into the inferior vena cava. The functional unit of the kidney is the nephron. Each kidney has been reported to contain between 600,000 and 1.5 million nephrons.323 The number of nephrons that an individual is born with (the “nephron dose”) may determine that individual’s susceptibility to renal injury. The nephron consists of a glomerulus, proximal tubule, loop of Henle, distal tubule, and collecting duct (see Figure 48-2). The collecting ducts ultimately combine to develop into the renal calyces, where the urine collects before passing along the ureter and into the bladder. The kidney is divided into several lobes. The outer, darker region of each lobe, the cortex, consists of most of the glomeruli and the proximal and distal tubules. The cortex surrounds a paler inner region, the medulla, which is further divided into a number of conical areas known as the renal pyramids, the apex of which extends toward the renal pelvis, forming papillae. Medullary rays are visible striations in the renal pyramids that connect the kidney cortex with the medulla. They are composed of descending (straight proximal) and ascending (straight distal) thick limbs of Henle and collecting ducts and associated blood vessels (the vasa recta). The central hilus is where blood vessels, lymphatics, and the renal pelvis (containing the ureter) join the kidney. The glomerulus is formed from a specialized capillary network. Each capillary develops into approximately 40 glomerular loops around 200 µm in size and consisting of a variety of different cell types supported on a specialized basement membrane (Figure 48-3, top). Some endothelial and epithelial cells act in concert with the specialized glomerular basement membrane (GBM) to form the glomerular filtration barrier, in addition to mesangial cells. Figure 48-3 The glomerular cells and the glomerular filtration barrier. A, Longitudinal section through a glomerulus and its juxtaglomerular apparatus. The capillary tuft consists of a network of specialized capillaries, which are outlined by a fenestrated endothelium (E). At the vascular pole, the afferent arteriole (AA) enters, branching into capillaries immediately after its entrance; the efferent arteriole (EA) is established inside the tuft and passes through the glomerular stalk before leaving at the vascular pole. The capillary network and the mesangium are enclosed in a common compartment bounded by the glomerular basement membrane (GBM). Note that there is no basement membrane at the interface between the capillary endothelium and the mesangium. The glomerular visceral epithelium consists of highly branched podocytes (POs), which, in a typical interdigitating pattern, cover the outer aspect of the GBM. At the vascular pole, the visceral epithelium and the GBM are reflected into the parietal epithelium (PE) of Bowman’s capsule, which passes over into the epithelium of the proximal tubule (PT) at the urinary pole. At the vascular pole, the glomerular mesangium is continuous with the extraglomerular mesangium (EGM), which consists of extraglomerular mesangial cells and an extraglomerular mesangial matrix. The EGM and the granular cells (G) of the afferent arteriole, along with the macula densa (MD), establish the juxtaglomerular apparatus. All cells that are suggested to be of smooth muscle origin are shown in black. US, Urinary space; F, foot processes; N, sympathetic nerve terminals; M, messenger cells. The capillary endothelial cells are about 40 nm thick and are in contact with each other. However, in contrast to the continuous endothelial linings seen elsewhere in the body, circular fenestrations (pores) with diameters of approximately 60 nm collectively constitute 20 to 50% of the glomerular endothelial surface.170 The endothelium permits virtually free access of plasma and small solutes to the basement membrane. However, although the fenestrations are far larger than the diameter of albumin (3.5 nm), it is thought that perm selectivity to such larger molecules begins at the endothelium because of the endothelial surface lining—a glycocalyx coating of negatively charged glycoproteins, glycosaminoglycans, proteoglycans, and absorbed plasma proteins, including orosomucoid and albumin. Estimates of the thickness of this layer vary depending on the visualization and preparation techniques used, but it may be between 200 and 400 nm thick.170 The basement membrane (Figure 48-3, bottom) of the glomerular capillaries is much thicker (approximately 300 nm) than that of other vascular beds and consists of three distinct electron-dense layers: the lamina rara interna, the lamina densa, and the lamina rara externa. The lamina densa consists of a close feltwork of fine, mainly type IV, collagen fibrils (each 3 to 5 nm thick) embedded in a gel-like matrix of laminin, nidogen/entactin, glycoproteins, and proteoglycans such as agrin and perlecan. The lamina densa forms the main size discriminant barrier to protein passage into the tubular lumen. The other two layers of the basement membrane are rich in negatively charged polyanionic glycoproteins, such as heparan sulfate; these may form a charge discriminant barrier to the passage of proteins, although the importance of the GBM in charge discrimination is still uncertain.170 The epithelial cells of the glomerulus line the outside of the glomerular capillaries, thus facing Bowman’s capsule and the primary urine (see Figure 48-3, top). These cells are called podocytes and have an unusual octopus-like structure in that they have a large number of cytoplasmic extensions or foot processes that are embedded in the basement membrane. Foot processes are anchored to the GBM via integrin molecules and dystroglycans and are divided into primary and secondary. The secondary processes between adjacent cells interdigitate to form filtration slits, which are 25 to 60 nm wide.170 The podocytes are covered by a complex diaphragm (“slit diaphragm”), some of the molecular components of which (e.g., nephrin) appear crucial for the maintenance of larger proteins within the circulation. The resulting structure is relatively impermeable to most proteins above 60 kDa, but passage of proteins is modulated by their charge and shape. Podocytes are also covered by a glycocalyx of sulfated molecules, including glycosaminoglycans and glycoconjugates (e.g., podocalyxin). The surface anionic charge helps to maintain the foot process structure and the distance between the parietal and visceral epithelial cells constituting Bowman’s space.170 The final cellular components of the glomerulus are the mesangial cells, which are found in the central part (“stalk”) of the glomerulus between and within the capillary loops suspended in a matrix that they synthesize. They are in direct contact with glomerular endothelial cells and the inner layer of the GBM (lamina rara interna), and also with the extraglomerular mesangium and the JGA (see Figure 48-3, top). Mesangial cells have the characteristics of smooth muscle cells (pericytes), in that they are rich in microfilaments and respond to and produce a variety of stimuli [e.g., angiotensin II (AII) and antidiuretic hormone (ADH) or arginine vasopressin].391 Mesangial matrix is rich in collagens and proteoglycans but is different in composition from the matrix of the GBM. Its composition and volume are tightly regulated in health but can be markedly altered during certain diseases [e.g., diabetic nephropathy, immunoglobulin (Ig)A nephropathy]. Mesangial cells have both structural and housekeeping functions. They have anchoring filaments to GBM opposite the podocytes, and their contractile properties enable them to alter intraglomerular capillary flow and glomerular ultrafiltration surface area, and thereby single nephron glomerular filtration rate (GFR). The cells appear to respond to capillary stretch by generating soluble factors such as vascular endothelial growth factor and transforming growth factor-β (TGF-β), and by activating intracellular signaling pathways. Mesangial cells also have specific and nonspecific mechanisms for removing macromolecules that reach the mesangial and subendothelial space, preventing their accumulation. These mechanisms include phagocytosis and degradation by the cells and trafficking along the mesangial stalk to the juxtaglomerular region, followed by elimination via the renal lymphatics, or by regurgitation into the glomerular capillary. Bowman’s capsule forms the beginning of the tightly coiled, proximal convoluted tubule (pars convoluta), which on its progress toward the renal medulla becomes straightened and is then called the pars recta. The proximal tubule is about 15 mm long. Epithelial cells lining the convoluted section are cuboidal/columnar cells with a luminal brush border consisting of millions of microvilli, which expand the surface area for absorption of tubular fluid. The proximal tubule is the most metabolically active part of the nephron (Table 48-1). The pars recta drains into the descending thin loop of Henle, which, after passing through a hairpin loop, becomes first the thin ascending limb, and then the thick ascending limb. The cells of the thin ascending limb are very similar to those in the descending (with little brush border, flattened and interdigitated), but important differences are evident in their permeability to water and in their capability for active transport. The thick ascending limb is lined with cuboidal/columnar cells similar in size to those in the proximal tubule, but they do not possess a brush border. At the end of the thick ascending limb, near where it re-enters the cortex and is closely associated with the glomerulus and the efferent arteriole, a cluster of cells known as the macula densa is present (Figure 48-4; see later). The main role of the loop of Henle is to assist in generating concentrated urine, hypertonic with respect to plasma; it also has several other functions (see Table 48-1). Where the thick ascending limb of the loop of Henle passes very close to the glomerulus of its own nephron, the cells of the tubule and the afferent arteriole show regional specialization (see Figure 48-4). The tubule forms the macula densa; the arteriolar cells are filled with granules (containing renin or its inactive precursor, prorenin) and are innervated with sympathetic nerve fibers. This area, called the JGA, plays an important part in maintaining systemic blood pressure through regulation of the circulating intravascular blood volume and sodium concentration via the renin-angiotensin-aldosterone system (RAAS). The proteolytic enzyme renin is released primarily in response to decreased afferent arteriolar pressure and decreased intraluminal sodium delivery to the macula densa. Renin is an enzyme of the hydrolase class that catalyzes cleavage of the leucine-leucine bond in angiotensinogen to generate angiotensin I. Renin release from the macula densa is also influenced by nitric oxide, renal cortical prostaglandins (predominantly PGI2), and the sympathetic nervous system. Angiotensin I is converted in the lungs by angiotensin-converting enzyme (ACE) to the potent vasoconstrictor and stimulator of aldosterone release, AII. Vasoconstriction and aldosterone release (with increased distal tubular sodium retention) act in concert with the other action of AII to increase the release of the nonapeptide ADH, and to increase proximal tubular sodium reabsorption, intravascular volume, and pressure. AII also has an inhibitory effect on renin release as part of a negative feedback loop. In a normal renal cortex, the interstitium is sparse (7 to 9% by volume) because the tubules lie very close together; however, a large proportion of the reabsorbed tubular fluid has to traverse a true interstitial space before entering the capillaries. The interstitium contains a variety of cell types, including lymphocytes and fibroblast-like cells.361 The kidneys regulate and maintain the constant optimal chemical composition of the blood and interstitial and intracellular fluids throughout the body—the internal milieu—through integration of the major renal functions, namely, filtration, reabsorption, and excretion. Mechanisms of differential reabsorption and secretion, located in the tubule of a nephron, are the effectors of regulation (Table 48-2). TABLE 48-2 Important Components of Kidney Function Transport of solutes and water occurs both across and between the epithelial cells that line the renal tubules. Transport is both active requiring energy and passive, but many of the so-called passive transport processes are dependent upon or secondary to active transport processes, particularly those involving sodium transport. All known transport processes involve receptor or mediator molecules, the activity of many of which is regulated by phosphorylation facilitated by protein kinase C or A. Their renal distribution has been shown to correlate with known regional functional activities, but the same transporters, or isoforms of them, can be found in other tissues, particularly the digestive tract. For instance, at least five independent proximal tubular transport processes may be noted for amino acids, including those for (1) basic amino acids plus cystine, (2) glutamic and aspartic acid, (3) neutral amino acids, (4) imino amino acids, and (5) glycine.489 Inherited disorders of tubular transporters, discussed later in this chapter, may occur, as well as a well-known generalized disorder affecting all of the transport processes, causing Fanconi syndrome and resulting in decreased reabsorption of electrolytes and nutrients (e.g., glucose, amino acids). Direct coupling of adenosine triphosphate (ATP) hydrolysis is an example of an active transport process. The most important enzymatic transporter in the nephron is Na+ K+-ATPase, which is located on the basolateral membranes of the tubuloepithelial cells, accounts for much of the renal oxygen consumption, and drives more than 99% of renal sodium reabsorption (Figure 48-5). Other examples of primary active transport mechanisms include a calcium-ATPase, an H+-ATPase, and an H+,K+-ATPase. These enzymes establish ionic gradients, polarizing cell membranes and thus driving secondary transport processes. Different regions of the tubule have been shown to specialize in certain functions. The proximal tubule facilitates the reabsorption of 60 to 80% of the glomerular filtrate volume, including 70% of the filtered load of sodium and chloride, and most of the potassium, glucose, bicarbonate, calcium, phosphate, sulfate, and other ions—secreting 90% of the hydrogen ion excreted by the kidney (see Table 48-1). Glucose is virtually completely reabsorbed, predominantly in the proximal tubule, by a passive but sodium-dependent process that is saturated at a blood glucose concentration of about 180 mg/dL (10 mmol/L). Uric acid is also reabsorbed in the proximal tubule by a passive sodium-dependent mechanism, but an active secretory mechanism is present. Creatinine is secreted but only to a small extent—approximately 2.5 µmol/min. Sodium reabsorption is required for the reabsorption of water and many solutes. The proximal tubule is highly permeable to sodium, and the net flux of reabsorption from the tubular lumen is achieved against a high backflux, particularly from paracellular* movement. Approximately 60% of filtered sodium is reabsorbed in the proximal tubule in an energy-dependent manner, driven by basolateral Na+,K+-ATPase pumps. Approximately 80% of sodium entering proximal tubular cells does so in exchange for hydrogen ion secretion, facilitated by apical Na-H exchangers. This process in turn permits bicarbonate reabsorption via carbonic anhydrases that are present in both the brush border and the intracellular compartment. A variety of apical sodium cotransporters also allow for reabsorption of other organic and inorganic solutes (e.g., chloride, calcium, phosphates, bicarbonate, sulfates,406 glucose, urea, amino acids). Sodium transport activity is regulated by many factors, including protein kinase–dependent phosphorylation, which can increase both activity and channel numbers. A further 30% of filtered sodium is reabsorbed in the thick ascending limb of the loop of Henle, where it is achieved by an apical, bumetanide-sensitive, 130 kDa, electroneutral, Na-K-2Cl cotransporter (NKCC2), itself driven by a favorable inward gradient generated by the basolateral Na+,K+-ATPase pump (Figure 48-6). NKCC2 is a kidney-specific member of a class of such channels found throughout secretory epithelia. Activation of these cotransporters appears, in part, to be a result of cell shrinkage. The distal tubule reabsorbs 5 to 8% of sodium via the apical thiazide-sensitive Na-Cl cotransporter (NCCT). Final sodium balance is achieved in the collecting duct via selective amiloride-sensitive, apical sodium channels (ENaCs) in exchange for potassium. ENaCs are controlled in part by the effects of aldosterone on the mineralocorticoid receptor (Figure 48-7). Figure 48-6 Schematic diagram showing the major pathways of solute reabsorption in the thick ascending limb of the loop of Henle. Sodium chloride is reabsorbed by the apical NKCC2 transporter. This electroneutral transport is driven by the low intracellular sodium and chloride concentrations generated by the basolateral Na+,Ka+-ATPase and the basolateral chloride channel CLC-Kb. The availability of potassium is rate-limiting for NKCC2, so potassium entering the cell is recycled back to the lumen via the ROMKI potassium channel. This potassium movement is electrogenic and drives paracellular resorption of Mg2+ and Ca2+ via paracellin-1. Mutations in NKCC2, ROMK1, or CLC-Kb cause Bartter’s syndrome. Mutations in paracellin-1 lead to disruption of this paracellular pathway and the tubular disease known as hypomagnesemic hypercalciuric nephrolithiasis.3 (From Sayer JA, Pearce SHS. Diagnosis and clinical biochemistry of inherited tubulopathies. Ann Clin Biochem 2001;38:459-70.) Approximately 90% of daily potassium loss occurs via renal elimination. Potassium is freely filtered across the glomerulus and normally is almost completely reabsorbed in the proximal tubule. However, most regulatory mechanisms affect the loop of Henle, the distal tubule, and the collecting duct. Indeed, urinary losses can exceed filtered load, indicating the importance of distal secretion. Determinants of urinary potassium loss are dietary intake of potassium and plasma potassium concentration, acid-base disturbances (acidosis reduces potassium secretion and vice versa), circulating ADH concentration (ADH increases potassium loss119), tubular flow rate (increased flow rate increases potassium loss240), and aldosterone secretion (enhances potassium loss and increases sodium retention).153 Potassium ions are actively accumulated within tubular cells as a result of basolateral Na+,K+-ATPase activity, resulting in elevation of intracellular potassium concentration to above its electrochemical equilibrium. Several types of potassium channels exist that have a number of functions: (1) maintenance of a negative resting cell membrane potential, (2) regulation of intracellular volume, (3) recycling of potassium across apical and basolateral membranes to supply NKCC2 and enable sodium reabsorption, and (4) potassium secretion in the cortical collecting tubule.32 As mentioned previously, potassium is reabsorbed with sodium by NKCC2 in the thick ascending limb of the loop of Henle, but it is recycled back into the lumen by the potassium-secreting channel, ROMK1, thus generating an electrical gradient that drives passive paracellular reabsorption of calcium and magnesium down their electrochemical gradient3 (see Figure 48-6). ROMK1 is a pH-sensitive, membrane-spanning protein with several serine residues. At least two of these residues require phosphorylation by protein kinase A for the channel to be active.32 In the principal cells of the collecting duct, sodium reabsorption via ENaC is accompanied by movement of potassium into the lumen through potassium channels or through a K-Cl symporter* (see Figure 48-7). Approximately 60% of chloride is reabsorbed in the proximal tubule. In the early part of the proximal tubule, avid reabsorption of sodium in combination with glucose and amino acids occurs, creating a lumen-negative potential difference. The negative potential difference drives chloride reabsorption by diffusion through the paracellular pathway. Preferential reabsorption of glucose, amino acids, and bicarbonate in association with sodium in the early proximal tubule causes an increase in the luminal chloride concentration. This high chloride composition heralds the second phase of proximal chloride (and sodium) reabsorption: passive diffusion of sodium chloride via the paracellular pathway, and active reabsorption involving several antiporter† systems, by which chloride is exchanged for secretion of other anions (e.g., bicarbonate, formate, oxalate). In the thick ascending limb of the loop of Henle, further chloride reabsorption occurs in association with sodium via NKCC2. The concentration gradient is maintained by a basolateral chloride pump, CLC-Kb (see Figure 48-6).239 Approximately 98% of filtered calcium is reabsorbed: 65 to 75% in the proximal tubule (via a paracellular pathway), 20 to 25% in the thick ascending limb of the loop of Henle, 10% in the distal tubule, and, finally, small amounts in the collecting ducts. Calcium reabsorption is predominantly a passive process linked to active sodium reabsorption. For example, in the thick ascending limb of the loop of Henle, paracellular calcium transport is driven by the potential difference created by ROMK1. Active processes, particularly in the distal tubule, tightly regulate the final amount of calcium excreted. Here, calcium reabsorption is transcellular, occurring against the existing electrochemical gradient, and is stimulated by parathyroid hormone (PTH). Following entry into the cell from the lumen via an apical epithelial active transport mechanism (ECaC1), calcium binds to calbindin-D and is delivered to the basolateral membrane. Here it is extruded by a calcium-ATPase (PMCA1b) and an Na-Ca exchanger (NCX1). Transcription of messenger RNA coding for both ECaC1 and calbindin is stimulated by calcitriol [1,25(OH2)D3], possibly synthesized locally in the distal nephron and acting in a paracrine and autocrine fashion. A functional vitamin D response element has been identified in the promoter region of the calbindin-D gene, along with a putative site in the ECaC1 gene. ECaC1 is a pH-sensitive, 83 kDa protein with six transmembrane-spanning domains. Activation of the ion channel probably involves protein kinase C phosphorylation. Evidence indicates that stimulation of the renal calcium-sensing receptor by calcium in the tubular lumen can directly affect tubular reabsorption of calcium, independent of the effects of calciotropic hormones.187 Reabsorption of phosphate occurs predominantly in the proximal tubule and is mediated by a secondary active transport mechanism. Three families (types I, II, and III) of sodium-dependent, phosphate cotransporters have been identified, of which type IIa (NPT2a, SLC34A1), a 640 amino acid protein located in the apical plasma membrane, is thought to be the most physiologically important. NPT2a sodium-phosphate transporter is electrogenic (i.e., involves the inward flux of a positive charge), with three sodium ions and one phosphate ion (preferentially divalent) being transferred. Acute regulation of transport is achieved primarily by an alteration in the amount of NPT2a protein present in the apical membrane, with longer-term changes also involving increased transcription of the protein [e.g., in response to 1,25(OH2)D3]. Tonic amounts of NPT2a in the apical membrane are thought to be high, with regulation predominantly involving internalization of the protein. Increased intracellular movement of the channel from the plasma membrane to the lysosomes is believed to follow both protein kinase A and C phosphorylation initiated by PTH receptor binding.429,430 Fibroblast growth factor 23 (FGF-23), is a 32 kDa phosphate-regulating peptide, largely produced by bone cells. It was discovered during the 1990s following studies of severe hereditary osteomalacia characterized by severe hypophosphatemia and inappropriate phosphaturia.56 Its major action is the inhibition of sodium-coupled reabsorption of inorganic phosphate in the renal proximal tubule. Autosomal dominant hypophosphatemic rickets is due to a mutation in the FGF-23 gene that results in a hyperstable form of this protein.26 The current paradigm suggests that phosphate ingestion and/or hyperphosphatemia causes FGF-23 release into the circulation from skeletal osteocytes and osteoblasts and interacts with receptors within the kidney via a transmembrane protein, klotho, thereby inhibiting the sodium-coupled phosphate cotransporter in the proximal tubule (Na-Pi type IIa, or NPt2a) and causing phosphaturia. FGF-23 also inhibits 1α-vitamin D hydroxylase, leading to reduced calcitriolproduction. These effects will reduce plasma phosphate concentrations. FGF23 may act in concert with PTH by reducing expression of NPT2a at the proximal tubule brush border, hence promoting phosphaturia.196 Efflux of phosphate across the basolateral membrane may involve an anion-exchange mechanism and/or a phosphate leak. Normally, less than 20% of the filtered load of phosphate is excreted into the urine, but above a plasma phosphate concentration of approximately 3.6 mg/dL (1.2 mmol/L), increments in urinary phosphate excretion increase linearly with the filtered load, suggesting that there is Tm (tubular maximal uptake) for phosphate. The Tm for phosphate is decreased by increases in the circulating PTH concentration and the ratio of Tm for phosphate to GFR (TmP/GFR). TmP/GFR has been used as a test in the differential diagnosis of hypercalcemia. Although superseded in this context by modern PTH assays, it may be useful in the investigation of inherited disorders of tubular phosphate handling.340 At least 11 different mammalian AQPs have been identified, of which seven (AQP1, -2, -3, -4, -6, -7, -8) are expressed in the kidney.320,321 Many of these have extrarenal expression sites as well (e.g., AQP1 may be important in fluid removal across the peritoneal membrane). Two asparagine-proline-alanine sequences in the molecule are thought to interact in the membrane to form a pathway for water translocation. AQP1, which is found in the proximal tubule and the descending thin limb of the loop of Henle, constitutes almost 3% of total membrane protein in the kidney. It appears to be constitutively expressed and is present in both the apical and basolateral plasma membranes, representing entry and exit ports for water transport across the cell, respectively. Approximately 70% of water reabsorption occurs at this site, predominantly via a transcellular (e.g., AQP1) rather than a paracellular route. Water reabsorption in the proximal tubule passively follows sodium reabsorption, so that fluid entering the loop of Henle is almost isosmotic with plasma. Urinary concentration is predominantly achieved by countercurrent multiplication in the loop of Henle (Figure 48-8).364,383 The descending thin limb is very permeable to water, but the ascending limb and the collecting duct are not (the collecting ducts are also poorly permeable to urea). Fluid entering the loop of Henle is isotonic to plasma but is hypotonic on leaving it. The ascending limb has active sodium reabsorption driven by Na+,K+-ATPase with electroneutralizing transport of chloride—a combined process that can be inhibited by the so-called loop diuretics (e.g., furosemide; see discussion later in this chapter). In this section of the nephron, sodium reabsorption is not accompanied by water, creating a hypertonic medullary interstitium and facilitating water reabsorption from the anatomically adjacent descending limb. The descending limb cells are permeable to sodium chloride, which is cycled from the descending limb back to the ascending limb. Continuous flow along the loop generates an osmotic gradient at the tip of the loop that can reach 1400 mOsmol/kg H2O. Approximately 5% of water is reabsorbed in the loop of Henle. A further 10% of water reabsorption occurs in the distal tubule, with the remainder (>20 L/d) reabsorbed in the collecting ducts. Entry of water into the collecting duct cells occurs via apical AQP2 channels, with exit probably occurring via basolateral AQP3 (cortical and outer medullary collecting ducts) and AQP4 (inner medullary collecting ducts). AQP2 appears to be the primary target for ADH regulation of water reabsorption. AQP2 is stored in subapical vesicles in the collecting duct cells. In response to ADH stimulation, these vesicles are cycled through, and inserted into, the plasma membrane by a cytoskeletal, dynein-mediated transport process. Stimulation occurs following binding of ADH to a V2 receptor in the basolateral plasma membrane of the principal cells of the collecting duct, which promotes a cyclic adenosine monophosphate (cAMP)/protein kinase A cascade, resulting in phosphorylation and activation of AQP2. ADH regulates the acute cellular water-retaining response (AQP2 trafficking) and its longer-term regulation via a conditioning effect on AQP2 gene transcription. The AQP2 gene has a cAMP response element that is involved in the long-term upregulation of AQP2 expression by ADH. It is likely that there are also ADH-independent regulatory pathways of AQP2 expression. Membrane insertion of AQP2 allows water to pass into the collecting duct cells under the influence of medullary hyperosmolality. Maintenance of medullary hyperosmolality depends upon efficient fluid removal, which is the function of the ascending vasa recta, a specialized medullary vasculature, and the close anatomic relations of all medullary constituents (see Figure 48-2). AQP2 expression is decreased in a variety of polyuric conditions (e.g., diabetes insipidus, lithium treatment, hypokalemia, hypercalcemia, urinary obstruction) and is increased in some water-retaining states (e.g., heart failure, cirrhosis, pregnancy).321,396 A variety of V2 receptor antagonists have been designed that block the actions of ADH. In contrast to diuretics, these agents promote the excretion of electrolyte-free water and have exciting therapeutic potential in water-retaining states.396 The endocrine functions of the kidneys may be regarded as primary, because the kidneys are endocrine organs producing hormones, or as secondary, because the kidneys are a site of action for hormones produced or activated elsewhere. In addition, the kidneys are a site of degradation for hormones such as insulin and aldosterone. In their primary endocrine function, the kidneys produce erythropoietin (EPO), prostaglandins and thromboxanes, 1,25(OH2)D3, and renin. The importance of renin in the maintenance of systemic blood pressure was discussed previously (see earlier, “Juxtaglomerular Apparatus”). Prostaglandins and thromboxanes are synthesized from arachidonic acid by the cyclooxygenase (COX) enzyme system (see Chapter 27 for further details). The COX system is present in many parts of the kidney and has an important role in regulating the physiologic action of other hormones on renal vascular tone, mesangial contractility, and tubular processing of salt and water. Prostaglandins have a critical role in renal hemodynamics, control of tubular function, and renin release. The major renal vasodilatory prostaglandin is PGE2, which is synthesized predominantly in the medulla. The major vasoconstrictor prostaglandin is thromboxane A2, which is produced primarily within the renal cortex.101 PGE2 increases renal blood flow rate, inhibits sodium reabsorption in the distal nephron and collecting duct, and stimulates renin release.101 These actions promote natriuresis and diuresis. In patients with chronic kidney disease (CKD), renal PGE2 production is increased, representing a compensatory response to loss of nephron mass.438 Vasodilatory prostaglandins are synthesized following stimulation with renal sympathetic adrenergic and AII-dependent mechanisms to offset or modulate vasoconstriction.295 In the tubule, prostaglandins act as autocoids, exerting their effects locally, near the site of synthesis. The kidneys are primarily responsible for producing 1,25(OH2)D3 from 25-hydroxycholecalciferol as a result of the action of the enzyme 25-hydroxycholecalciferol 1α-hydroxylase found in proximal tubular epithelial cells. Regulation of this system is discussed in Chapter 52. The management of renal osteodystrophy is considered later in this chapter. The GFR is considered to be the most reliable measure of the functional capacity of the kidneys and is often thought of as indicative of the number of functioning nephrons. As a physiological measurement, it has proved to be the most sensitive and specific marker of changes in overall renal function. Measurement of GFR is discussed in Chapter 25. Kf = (hydraulic permeability × surface area) PGCap = glomerular-capillary hydrostatic pressure ΠBC = oncotic pressure in Bowman’s capsule PBC = hydrostatic pressure in Bowman’s capsule The factors involved in regulation of GFR are listed in Table 48-3. Autoregulation of renal blood flow and GFR is widely thought to be explained by the myogenic theory. This theory is based on the principle that an increase in wall tension of the afferent arterioles, brought about by an increase in perfusion pressure, causes automatic contraction of arteriolar smooth muscle, thus increasing resistance and keeping the flow constant despite the increase in perfusion pressure. TABLE 48-3 The tubuloglomerular feedback mechanism, involving the macula densa and release of the vasodilator adenosine, must also be considered. Although not fully understood, this mechanism appears to regulate GFR, with changes in renal blood flow as a secondary consequence. For individual nephrons, evidence indicates that each single nephron GFR (SNGFR) is influenced by the composition of the tubular fluid in the distal tubule, which in turn is influenced by the filtration rate. The macula densa is thought to sense the distal tubular sodium chloride content, its osmolality, or the rate at which sodium chloride is transported. The macula densa then signals the JGA via an uncertain mechanism to cause the release of adenosine and possibly AII and prostaglandins, which in turn affects vascular resistance.* Other factors influencing renal blood flow are indicated in Table 48-4. The afferent and efferent arterioles are richly supplied with renal sympathetic nerves. Epinephrine acts via α-adrenergic receptors, leading to constriction of both arterioles and causing a decrease in renal blood flow. TABLE 48-4 Factors Altering Renal Artery Tone and Renal Blood Flow GFR, Glomerular filtration rate; N, negative; NE, negligible; P, positive; RBF, renal blood flow. Nitric oxide (NO) has been identified as an important vasodilator produced by vascular endothelial cells. NO is synthesized from L-arginine and oxygen by nitric oxide synthetase (NOS), of which three isoenzymes are differentially located and regulated. Within the kidney are eNOS (endothelial) and iNOS (inducible) isoenzymes. Activation of NOS has been shown to occur as a result of shear stress (e.g., increased arteriolar tone). A variety of physiologic vasoconstrictors are present, including acetylcholine, bradykinin, endothelin, and serotonin; a rise in intracellular ionized calcium is required for the vasoconstrictors. NO synthesis is now known to play an important role in the regulation of human vascular tone and has a crucial role in control of blood pressure and kidney function.102,160,359 It has also been found in the macula densa and has been implicated in the regulation of renin release. Kidney function is not constant throughout life. In utero, urine is produced by the developing fetus from about the ninth week of gestation. Nephrogenesis is complete by approximately 35 weeks’ gestation, although kidney function remains immature during the first 2 years of life. The kidney of the term infant receives approximately 6% of the cardiac output, compared with 25% in adults. Renal vascular resistance is relatively high, and the low renal blood flow is particularly directed to the medulla and inner cortex. The gradual increase in renal blood flow that occurs with increasing age is directed mainly to the outer cortex and is mediated by local neurohormonal mechanisms.216 The GFR at birth is approximately 30 mL/min/1.73 m2.72 It increases rapidly during the first weeks of life to reach approximately 70 mL/min/1.73 m2 by age 16 days.72 Normal adult values are achieved by age 14 years. Tubular functions, including salt and water conservation, are also immature at birth. Birth is associated with rapid changes in kidney function, with a switch to salt and water conservation mediated by catecholamines, the renin-angiotensin system, ADH, glucocorticoids, and thyroid hormone.72 The immaturity of the neonatal kidney contributes to the relatively common problems of water and electrolyte disturbances in infants. These disturbances are more likely to occur in premature infants, particularly those born before 35 weeks’ gestation. Aging is associated with a variety of structural changes in the kidney, which begin in early middle age, including decreasing kidney weight and number of glomeruli, with the cortical glomeruli being particularly affected.110,222 Changes in the afferent and efferent arteriolar systems are evident, with formation of direct channels (shunts) between afferent and efferent arterioles in the medulla. Aging is also associated with the development of tubulointerstitial fibrosis, loss of tubular mass, and decreasing length of the proximal tubule. Structural change is accompanied by functional changes, which in many respects are the reverse of those seen in early life. On average, GFR declines with age by approximately 1 mL/min/1.73 m2/y over the age of 40 years.11,92,375,459 Renal blood flow, particularly to the cortical area, also decreases with age, while the filtration fraction (i.e., GFR/renal plasma flow)11 and renal vascular resistance123,124 increase. Tubular function, such as the ability to concentrate urine and excrete a water and salt load, is decreased, and nocturnal polyuria is common. Renal salt conservation is impaired,110 and the prevalence of albuminuria rises over the age of approximately 40 years.95,213 It is not known whether these changes are the result of a normal aging process (i.e., involutional) or whether they are caused by the interplay of pathology and age. Cumulative exposure to common causes of CKD such as (1) atherosclerosis, (2) hypertension, (3) heart failure, (4) diabetes,65 (5) obstructive nephropathy, (6) infection, (7) immune insult, (8) nephrotoxins such as lead,262 and (9) dietary protein5,47 increases with age, and it is difficult to separate these effects from those of “healthy” aging. The decline in GFR with increasing age may be largely attributable to hypertension,265,266 atherosclerosis,222 or heart failure.122 In the absence of these and other identifiable causes of kidney disease, many individuals have stable GFR as they age. Loss of kidney function with aging appears to be heterogeneous and is not inevitable.123,264 Kidney function may be well preserved in healthy older people, and assumptions with respect to GFR based solely on age could be erroneous. Conversely, attention to the common causes of CKD could preserve function in older people.395 Kidney disease is more common among older people. Studies from England,116 France,217 and Iceland281 have demonstrated a near exponential rise in CKD with age. Data from the United States on CKD prevalence show the prevalence of a GFR between 30 and 60 mL/min/1.73 m2 to be 4.3% of the total noninstitutionalized population overall, but this rises to 25% among those over 70 years.83 The prevalence may be even higher among institutionalized older people (e.g., 82% of a residential home population were identified as having a GFR <60 mL/min/1.73 m2).61 The incidence of acute kidney injury also increases with age.230 Glomerular permselectivity to proteins is a function of the integrated actions of endothelial cells, the GBM, and the podocytes, although the exact contribution and importance of each is still a matter of some debate. A variety of methods have been used to study the permeability of the glomerular barrier, including urinalysis in vivo, micropuncture of single nephrons, isolated perfused kidneys, isolated glomeruli, isolated GBMs, and artificial membranes. All of these techniques have contributed to knowledge of glomerular permeability characteristics, and all have advantages and disadvantages. For example, micropuncture techniques may damage the barrier. These issues have been reviewed by Haraldsson and associates.170 Additionally, a number of different markers, including endogenous and modified proteins, dextran, and Ficoll polymers, have been used to study glomerular permeability.170 The glomerular permeability of a molecule is expressed in terms of its glomerular sieving coefficient (GSC). Molecules smaller than approximately the molecular weight of inulin (5 kDa) are freely filtered. Therefore, inulin, urea, creatinine, glucose, and electrolytes all have a GSC = 1.0. Classic experiments in the 1970s used linear dextran chains of varying molecular weight and charge to study glomerular filtration characteristics. However, linear carbohydrate chains do not necessarily behave in the same manner as a globular protein of equal molecular weight or charge. For example, neutral dextran chains of 15 kDa (diameter 2.4 nm) have GSC = 1.0, whereas the smaller β2-microglobulin (11.8 kDa, diameter 1.6 nm) has GSC = 0.7.24 Linear molecules have higher GSC than globular proteins, hence theoretical glomerular pore dimensions based on dextran studies were overestimated. More recently, Ficoll polymers have been used. These are neutral, heavily cross-linked, sucrose-epichlorohydrin copolymers that behave as rigid hydrated spheres and are thought to behave more like globular proteins in their sieving behavior.170 As a result of such studies, some general conclusions can be drawn with respect to glomerular protein handling. The glomerulus acts as a selective filter of the blood passing through its capillaries, restricting the passage of macromolecules in a size-, charge-, and shape/configuration-dependent manner. Sieving coefficients (1) decrease as molecular size increases, (2) are lower for anionic proteins than for neutral proteins of equivalent size, and (3) are lower for globular rather than elongated proteins. Examples of the GSC for major urinary proteins are listed in Table 25-2. The protein concentration in the glomerular filtrate has been measured in several animal models by direct glomerular puncture. The concentration of total protein found is in the range of several hundred mg/L (≈1% of plasma), with albumin concentrations varying from less than 40 to a few hundred mg/L. The filtered load of protein depends on the product of the GSC and the free plasma concentration; therefore the albumin load per nephron is much greater than that of the other filtered proteins.24,275 In general, proteins larger than albumin (66 kDa, diameter 3.5 nm, charge −23) are retained by the healthy glomerulus and are termed high molecular weight proteins. However, lower molecular weight proteins are also retained to a significant extent. The final urinary concentration of proteins depends on the filtered load, but also on the efficiency of the proximal tubular reabsorptive process, in addition to any contribution of tubular secretion. Proteins are reabsorbed by receptor-mediated, low-affinity, high-capacity processes. Megalin (MW 600 kDa) and cubulin (MW 460 kDa) are endocytic, multiligand receptors that are important in protein reabsorption.455 Megalin belongs to the low-density lipoprotein (LDL) receptor family, whereas cubulin is identical to the intestinal intrinsic factor–vitamin B12 receptor. In the kidney, both are localized in clathrin-coated pits in the apical brush border of renal proximal tubular cells and bind filtered proteins in a calcium-dependent process. Megalin appears capable of both binding and internalizing its ligands, whereas the cubulin-ligand complex requires megalin to be internalized. Some proteins such as albumin will bind to either receptor, whereas others are specific [e.g., transferrin binds to cubulin only, retinol-binding protein (RBP) and α1-microglobulin to megalin only]. Once proteins have been internalized, they are transported by the endocytic vesicle and fuse with lysosomes. Proteolysis occurs, and the resultant amino acids are released into the tubulointerstitial space across the basolateral surface of the tubular epithelial cell. The membrane vesicles are then recycled to the brush border to complete the reabsorption cycle. In health, the reabsorptive mechanism removes 99% of the filtered protein, thus retaining most of the essential amino acid constituents for reuse.24,162,275 Capture of filtered transport proteins is also important in conserving vitamin status (e.g., vitamin A associated with RBP). Tubular secretion of proteins also contributes to urinary total protein concentration; in particular, in health, Tamm Horsfall glycoprotein (THG) accounts for ≈50% of urinary total protein. THG (MW 200 kDa), a highly glycosylated acidic protein, is secreted into the tubular fluid only by the thick ascending limb and the early distal convoluted tubule and is thought to play a role in inhibiting kidney stone formation.190,373 It is a major constituent of renal tubular casts, along with albumin and traces of other proteins. Investigation for increased urinary protein loss is mandatory in any patient with suspected kidney disease and was considered in Chapter 25. It is increasingly accepted that proteinuria is not just a marker of, but contributes directly to, progression of kidney disease.52,54 The accumulation of proteins in abnormal amounts in the tubular lumen may trigger an inflammatory reaction, which in turn may contribute to interstitial structural damage and expansion, and progression of kidney disease.51 Increasing evidence suggests that megalin may not just be a scavenger receptor for albumin, but that it may have signaling functions that regulate cell survival. Excessive quantities of albumin in the tubular lumen may downregulate proximal tubular megalin expression, increasing cell sensitivity to apoptosis.13 Evidence gathered from in vitro studies suggests that glomerular filtration of abnormal amounts or types of protein induces mesangial cell injury, leading to glomerulosclerosis, and that these same proteins can have adverse effects on proximal tubular cell function.105 Numerous studies have demonstrated that proteinuria is a potent risk marker for progression of renal disease in both nondiabetic202,346,377 and diabetic48,189 kidney disease. Furthermore, reducing proteinuria slows the rate of progression of proteinuric kidney disease. This effect has been observed in clinical trials in patients treated with ACE inhibitors and angiotensin II receptor blockers (ARBs), given alone or in combination.337,376 These drugs reduce protein excretion by reducing intraglomerular filtration pressure and possibly by stabilizing the glomerular epithelial cell slit diaphragm proteins.6,276 Consequently, reduction of proteinuria is an important therapeutic target.185,203,225
Kidney Disease
Anatomy
Blood Supply
Nephron
Glomerulus
B, Glomerular capillary wall. In glomerular filtration, filtered fluid is believed to traverse the capillary wall via an extracellular route, that is, through endothelial fenestrae, basement membrane, and slit diaphragms. Circulating polyanions (e.g., albumin) are thought to be retarded by the rich distribution in inner barriers of negatively charged sialylated glycoproteins (shaded area in schematic diagram). (A From Elger M, Kriz W. The renal glomerulus—the structural basis of ultrafiltration. In: Cameron JS, Davison AM, Grunfeld JP, Kerr D, Ritz E, eds. Oxford textbook of clinical nephrology, volume 1, 2nd edition. Oxford: Oxford University Press, 1998:Chapter 3.1. Reproduced by permission of Oxford University Press; B From Brenner BM, Beeuwkes R III. The kidney in health and disease: III. The renal circulations. Hosp Pract 1978;13:35-46.)
Proximal Tubule
Loop of Henle
Juxtaglomerular Apparatus
Renal Interstitium
Kidney Function and Physiology
Filtration
Preparation of an ultrafiltrate
Reabsorptive
Glucose, amino acids, electrolytes, proteins
Homeostatic
Extracellular volume, acid-base status, blood pressure, electrolytes
Metabolic
Synthetic: glutathione, glyconeogenesis, ammonia
Catabolic: hormones, cytokines
Endocrine
Erythropoietin synthesis, activation of vitamin D, renin release
Excretory and Reabsorptive Functions
Formation of Urine—An Overview
Regulatory Function
Sodium
Potassium
Chloride
Calcium
Phosphate
Water Homeostasis
Endocrine Function
Prostaglandins and Thromboxanes
1,25(OH2)D3
Glomerular Filtration Rate
Regulation of GFR
Major Influencing Factors
Effect on GFR
Kf
Increased glomerular surface area due to relaxation of mesangial cells
Increase
Decreased glomerular surface area due to contraction of mesangial cells
Decrease
PGCap
Altered renal arterial pressure
Afferent dilation
Increase
Afferent constriction
Decrease
Efferent constriction
Increase
Efferent dilation
Decrease
PBC
Increased intratubular pressure (e.g., tubular obstruction)
Decrease
ΠGCap
Altered plasma oncotic pressure: increased
Decrease
Altered renal blood flow: decreased
Decrease
Age and the Kidney
Glomerular and Tubular Protein Handling
Glomerular Sieving
Tubular Reabsorption
Consequences of Proteinuria
Stay updated, free articles. Join our Telegram channel
Full access? Get Clinical Tree
Kidney Disease
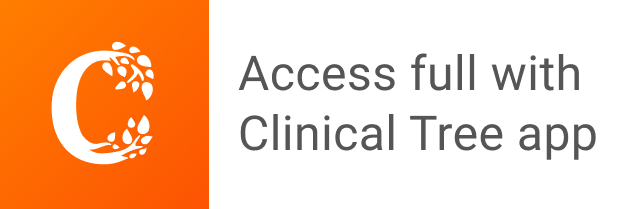