Chapter 12 Kidney and urinary tract disease
Functional anatomy
Renal arteries and arterioles
The renal artery undergoes a series of divisions within the kidney (Fig. 12.1) forming successively, the interlobar arteries, which run radially to the corticomedullary junction, arcuate arteries, which run circumferentially along the corticomedullary junction, and interlobular arteries, which run radially through the renal cortex towards the surface of the kidney. Afferent glomerular arterioles arise from the interlobular arteries to supply the glomerular capillary bed, which drains into efferent glomerular arterioles. Efferent arterioles from the outer cortical glomeruli drain into a peritubular capillary network within the renal cortex and then into increasingly large and more proximal branches of the renal vein. By contrast, blood from the juxtamedullary glomeruli passes via the vasa recta in the medulla and then turns back towards the area of the cortex from which the vasa recta originated.
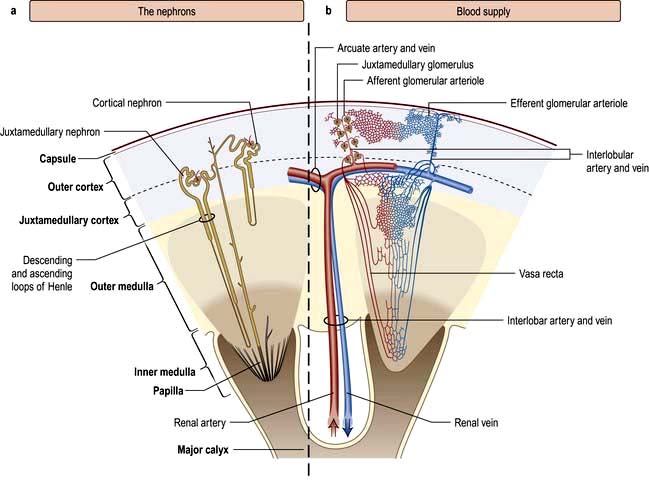
Figure 12.1 Functional anatomy of the kidney. (a) The nephrons. (b) Arterial and venous supply.
(After Standring S (ed) 2008 Gray’s Anatomy, 40th edn. Edinburgh: Churchill Livingstone).
The glomerulus
The glomerulus comprises four main cell types:
Endothelial cells which are fenestrated with 500–1000 Å pores
Visceral epithelial cells (podocytes) which support the delicate glomerular basement membrane by means of an extensive trabecular network (foot processes)
Parietal epithelial cells which cover the Bowman’s capsule
Mesangial cells are believed to be related to macrophages of the reticuloendothelial system and have a phagocytic function and contractile capabilities that can control blood flow and filtration surface area along the glomerular capillaries in response to a host of mediators. They also secrete the mesangial matrix, which provides a skeletal framework for the glomerular capillaries. The glomerular capillary basement membrane lies between the endothelial and the visceral epithelial cells. The latter put out multiple long foot processes which interdigitate with those of adjacent epithelial cells. Together the endothelial cells, basement membrane and epithelial cells form the filtration barrier or sieve (see Fig. 12.12a).
Renal tubules
The renal tubules are lined by epithelial cells, which are cuboidal except in the thin limb of the loop of Henle where they are flat. Proximal tubular cells differ from other cells of the system as they have a luminal brush border. The cortical portion of the collecting ducts contains two cell types with different functions, namely principal cells and intercalated cells (see p. 561). Fibroblast-like cells in the renal cortical interstitium have been shown to produce erythropoietin in response to hypoxia (p. 567).
The juxtaglomerular apparatus
The juxtaglomerular apparatus comprises the macula densa, the extraglomerular mesangium and the terminal portion of the afferent glomerular arteriole (which contains renin-producing granular cells) together with the proximal portion of the efferent arteriole. The macula densa is a plaque of cells containing large, tightly packed cell nuclei (hence the name macula densa; see Fig. 12.2) within the thick ascending limb of the loop of Henle. This anatomical arrangement is such as to allow changes in the renal tubule to influence behaviour of the adjacent glomerulus (tubuloglomerular feedback).
Renal function
Physiology
A conventional diagrammatic representation of the nephron is shown in Figure 12.2a and a physiological version in Figure 12.2b.
A hydrostatic pressure gradient of approximately 10 mmHg (a capillary pressure of 45 mmHg minus 10 mmHg of pressure within Bowman’s space and 25 mmHg of plasma oncotic pressure) provides the driving force for ultrafiltration of virtually protein-free and fat-free fluid across the glomerular capillary wall into Bowman’s space and so into the renal tubule (Fig. 12.3).
The ultrafiltration rate (glomerular filtration rate; GFR) varies with age and sex but is approximately 120–130 mL/min per 1.73 m2 surface area in adults. This means that, each day, ultrafiltration of 170–180 L of water and unbound small-molecular-weight constituents of blood occurs. If these large volumes of ultrafiltrate were excreted unchanged as urine, it would be necessary to ingest huge amounts of water and electrolytes to stay in balance. This is avoided by the selective reabsorption of water, essential electrolytes and other blood constituents, such as glucose and amino acids, from the filtrate in transit along the nephron. Thus, 60–80% of filtered water and sodium are reabsorbed in the proximal tubule along with virtually all the potassium, bicarbonate, glucose and amino acids (Fig. 12.2b). Additional water and sodium chloride are reabsorbed more distally, and fine tuning of salt and water balance is achieved in the distal tubules and collecting ducts under the influence of aldosterone and antidiuretic hormone (ADH). The final urine volume is thus 1–2 L daily. Calcium, phosphate and magnesium are also selectively reabsorbed in proportion to the need to maintain a normal electrolyte composition of body fluids.
Urine concentration and the countercurrent system
Urine is concentrated by a complex interaction between the loops of Henle, the medullary interstitium, medullary blood vessels (vasa recta) and the collecting ducts (see p. 640). The proposed mechanism of urine concentration is termed ‘the countercurrent mechanism’. The countercurrent hypothesis states that: ‘a small difference in osmotic concentration at any point between fluid flowing in opposite directions in two parallel tubes connected in a hairpin manner is multiplied many times along the length of the tubes’. Tubular fluid moves from the renal cortex towards the papillary tip of the medulla via the proximal straight tubule and the thin descending limb of the loop of Henle, which is permeable to water and impermeable to sodium. The tubule then loops back towards the cortex so that the direction of the fluid movement is reversed in the ascending limb, which is impermeable to water but permeable to sodium. This results in a large osmolar concentration difference between the corticomedullary junction and the hairpin loop at the tip of the papilla, and hence countercurrent multiplication. There is an analogy with heat exchangers.
Since the urine that emerges from the proximal tubule is iso-osmotic, the first nephron segment actually involved in urinary concentration is the descending limb of Henle’s loop. There are two types of descending limbs (Fig. 12.2b).
The short loops originate in superficial and midcortical glomeruli, and turn in the outer medulla. Approximately 85% of nephrons have these.
The long loops, which originate in the deep cortical and juxtamedullary glomeruli, comprise 15% of nephrons which penetrate the outer medulla up to the tip of the papilla.
Glomerular filtration rate (GFR)
The concentration of urea or creatinine in plasma represents the dynamic equilibrium between production and elimination. In healthy subjects there is an enormous reserve of renal excretory function, and serum urea and creatinine do not rise above the normal range until there is a reduction of 50–60% in the GFR. Thereafter, the level of urea depends both on the GFR and its production rate (Table 12.1). The latter is heavily influenced by protein intake and tissue catabolism. The level of creatinine is much less dependent on diet but is more related to age, sex and muscle mass. Once it is elevated, serum creatinine is a better guide to GFR than urea and, in general, measurement of serum creatinine is a good way to monitor further deterioration in the GFR.
Table 12.1 Factors influencing serum urea levels
Production | Elimination |
---|---|
Increased by | Increased by |
High-protein diet | Elevated GFR, e.g. pregnancy |
Increased catabolism | |
Surgery | Decreased by |
Infection | Glomerular disease |
Trauma | Reduced renal blood flow |
Corticosteroid therapy | Hypotension |
Tetracyclines | Dehydration |
Gastrointestinal bleeding | Urinary obstruction |
Cancer | Tubulointerstitial nephritis |
Decreased by |
|
Low-protein diet |
|
Reduced catabolism, e.g. old age |
|
Liver failure |
|
GFR, glomerular filtration rate.
It must be re-emphasized that a normal serum urea or creatinine is not synonymous with a normal GFR.
Measurement of the glomerular filtration rate
Measurement of the GFR is necessary to define the exact level of renal function. It is essential when the serum (plasma) urea or creatinine is within the normal range. The most widely used measurement is the creatinine clearance (Fig. 12.4).
Calculated GFR. Measurement of true GFR is cumbersome, time-consuming and may be inaccurate if 24-hour urine collections are incomplete. Therefore, several formulae have been developed that allow a prediction of creatinine clearance or GFR from serum creatinine and demographics. The Cockcroft–Gault equation for creatinine clearance is shown in Box 12.1.
Box 12.1
Estimation of glomerular filtration rate (GFR)
Modification of diet in renal disease (MDRD) equation
Calculation of estimated GFR by four variables:
To convert creatinine values in µmol/L to mg/dL multiply by 0.0113.
A prediction equation has been developed based on the data derived from the Modification of Diet in Renal Disease (MDRD) study in people with chronic kidney disease (CKD) (Box 12.1). This equation is based on age, sex, creatinine and ethnicity. A modification of MDRD equation is used by most chemical pathology laboratories to calculate eGFR but it is less reliable if actual GFR is >60 mL/min and can result in inappropriate referral to renal physicians.
Tubular function
The major function of the tubule is the selective reabsorption or excretion of water and various cations and anions to keep the volume and electrolyte composition of body fluid normal (see Ch. 13).
Conversely, inherited or acquired defects in tubular function may lead to incomplete absorption of a normal filtered load, with loss of the compound in the urine (a lowered ‘renal threshold’). This is seen in renal glycosuria, in which there is a genetically determined defect in tubular reabsorption of glucose. It is diagnosed by demonstrating glycosuria in the presence of normal blood glucose levels. Inherited or acquired defects in the tubular reabsorption of amino acids, phosphate, sodium, potassium and calcium also occur, either singly or in combination. Examples include cystinuria and Fanconi’s syndrome (see p. 1040 and Ch. 13). Tubular defects in the reabsorption of water result in nephrogenic diabetes insipidus (p. 992). Under normal circumstances, antidiuretic hormone induces an increase in the permeability of water in the collecting ducts by attachment to receptors with subsequent activation of adenyl cyclase. This then activates a protein kinase, which induces preformed cytoplasmic vesicles containing water channels (termed ‘aquaporins’) to move to and insert into the tubular luminal membrane. This allows water entry into tubular cells down a favourable osmotic gradient. Water then crosses the basolateral membrane and enters the bloodstream. When the effect of ADH wears off, water channels return to the cell cytoplasm (see Fig. 13.5).
Investigation of tubular function in clinical practice
Two tests of distal tubular function are commonly applied in clinical practice:
These tests are dealt with on page 993 and page 665.
Endocrine function
Renin-angiotensin system
Juxtaglomerular apparatus
Pressure changes in the afferent arteriole
Chloride and osmotic concentration in the distal tubule via the macula densa (Fig. 12.2a)
The renin-angiotensin-aldosterone system is illustrated in Figure 12.5.
causes rapid, powerful vasoconstriction
stimulates the adrenal zona glomerulosa to increase aldosterone production (over hours or days), leading to sodium and water retention.
In addition to influencing systemic haemodynamics, angiotensin II also regulates GFR. Although it constricts both afferent and efferent arterioles, vasoconstriction of efferent arterioles is three times greater than that of afferent, resulting in increase of glomerular capillary pressure and maintenance of GFR. In addition, angiotensin II constricts mesangial cells, reducing the filtration surface area, and sensitizes the afferent arteriole to the constricting signal of tubuloglomerular feedback (see p. 562). The net result is that angiotensin II has opposing effects on the regulation of GFR: (a) an increase in glomerular pressure and consequent rise in GFR; (b) reduction in renal blood flow and mesangial cell contraction, reducing filtration (see Fig. 12.48). In renal artery stenosis with resultant low perfusion pressure, angiotensin II maintains GFR. However, in cardiac failure and hypertension, GFR may be reduced by angiotensin II.
The renin-angiotensin system can be blocked at several points with renin inhibitors, angiotensin-converting enzyme inhibitors (ACEI) and angiotensin II receptor antagonists (A-IIRA). These are useful agents in treatment of hypertension and heart failure (see p. 782 and p. 719) but have differences in action: ACEIs also block kinin production while A-IIRAs are specific for the AT-II receptors.
Erythropoietin
Erythropoietin (see also p. 374) is the major stimulus for erythropoiesis. It is a glycoprotein produced principally by fibroblast-like cells in the renal interstitium.
Under hypoxic conditions both the α and β subunits of hypoxia inducible factor 2 (HIF-2) are expressed forming a heterodimer, causing erythropoietin gene transcription via the combined effects of hepatic nuclear factor 4 (HNF-4) and coactivator p300. Erythropoietin, once formed, binds to its receptors on erythroid precursor cells.
Under normal oxygen conditions, only the HIF-2-β subunit is constitutively expressed. The α subunit undergoes proline hydroxylation in the presence of iron and oxygen by prolyl hydroxylase.
The hydroxylated HIF-2-α subunit binds to von Hippel-Lindau protein and a ubiquitin ligase E3 complex is activated. This leads to ubiquitination (p. 31) and subsequent degradation of HIF-2-α via proteosomes so that no erythropoietin is transcribed. In normoxic conditions HIF-2-α also undergoes asparaginyl hydroxylation which prevents HIF complex from recruiting coactivators. These hydroxylation steps have absolute requirement for molecular oxygen which forms the basis of oxygen sensing.
Loss of renal substance, with decreased erythropoietin production, results in a normochromic, normocytic anaemia. Conversely, erythropoietin secretion may be increased, with resultant polycythaemia, in people with polycystic renal disease, benign renal cysts or renal cell carcinoma. Recombinant human erythropoietin has been biosynthesized and is available for clinical use, particularly in people with chronic kidney disease (CKD) (see p. 623).
Vitamin D metabolism
Naturally occurring vitamin D (see also p. 622) (cholecalciferol) requires hydroxylation in the liver at position 25 and again by a 1α-hydroxylase enzyme (mitochondrial cytochrome P450) mainly in the distal convoluted tubule, the cortical and inner medullary part of the collecting ducts and the papillary epithelia of the kidney to produce the metabolically active 1,25-dihydroxycholecalciferol (1,25-(OH)2D3). The 1α-hydroxylase activity is increased by high plasma levels of parathyroid hormone (PTH), low phosphate and low 1,25-(OH)2D3. 1,25-dihydroxycholecalciferol and 25-hydroxycholecalciferol are degraded in part by being hydroxylated at position 24 by 24-hydroxylase. The activity of this enzyme is reduced by PTH and increased by 1,25-(OH)2D3 (which therefore promotes its own inactivation).
Autocrine function
Prostaglandins
Prostaglandins are unsaturated, oxygenated fatty acids, derived from the enzymatic metabolism of arachidonic acid, mainly by constitutively expressed cyclo-oxygenase-1 (COX-1) or inducible COX-2 (see Fig. 15.30). COX-1 is highly expressed in the collecting duct, while COX-2 expression is restricted to the macula densa. Both COX isoforms convert arachidonic acid to the same product, the bioactive but unstable prostanoid precursor, prostaglandin H2 (PGH2). PGH2 is converted to:
PGE2 (formed by PDE2 synthase in the collecting duct, responsible for natriuretic and diuretic effects)
PGD2 (undetermined significance, produced in proximal tubule)
prostacyclin (PGI2) (mainly synthesized in the interstitial and vascular compartment)
thromboxane A2 (vasoconstrictor, mainly synthesized in glomerulus).
Nitric oxide and the kidney
Nitric oxide (see Fig. 16.18), a molecular gas, is formed by the action of three isoforms of nitric oxide synthase (NOS; p. 879). The most recognized cellular target of nitric oxide is soluble guanylate cyclase. The stimulation of this enzyme enhances the synthesis of cyclic GMP from GTP. All three isoforms are expressed in the kidney with eNOS in the vascular compartment, nNOS mainly in the macula densa and inner medullary collecting duct, and iNOS in several tubule segments. Nitric oxide mediates the following physiological actions in the kidney:
Regulation of renal haemodynamics
Natriuresis by inhibiting Na+/K+-ATPase and Na+/H+ antiporter and antagonizing ADH
Modulation of tubuloglomerular feedback so that the composition of tubular fluid delivered to the macula densa changes the filtration rate of the associated glomerulus.
Investigations
Examination of the urine
Chemical (Stix) testing
Blood
Haematuria may be overt, with bloody urine, or microscopic and found only on chemical testing. A positive Stix test must always be followed by microscopy of fresh urine (with the exception of menstruating women) to confirm the presence of red cells or red-cell casts and so exclude the relatively rare conditions of haemoglobinuria or myoglobinuria. Bleeding may come from any site within the urinary tract (Fig. 12.6):
Overt bleeding from the urethra is suggested when blood is seen at the start of voiding and then the urine becomes clear.
Blood diffusely present throughout the urine comes from the bladder or above.
Blood only at the end of micturition suggests bleeding from the prostate or bladder base.
Bacteriuria
Dipstick tests for bacteriuria are based on the detection of nitrite produced from the reduction of urinary nitrate by bacteria and also for the detection of leucocyte esterase, an enzyme specific for neutrophils. Although each test on its own has limitations, a positive reaction with both tests has a high predictive value for urinary tract infection (p. 593).
Microscopy
White blood cells. The presence of ≥10 WBCs/mm3 in fresh unspun mid-stream urine samples is abnormal and indicates an inflammatory reaction within the urinary tract such as urinary tract infection (UTI), stones, tubulointerstitial nephritis, papillary necrosis, tuberculosis and interstitial cystitis.
Red cells. The presence of one or more red cells per cubic millimetre in unspun urine samples results in a positive Stix test for blood and is abnormal.
Casts are cylindrical bodies, moulded in the shape of the distal tubular lumen, and may be hyaline, granular or cellular. Coarse granular casts occur with pathological proteinuria in glomerular and tubular disease. Red-cell casts – even if only single – always indicate renal disease. White cell casts may be seen in acute pyelonephritis. They may be confused with the tubular cell casts that occur in patients with acute tubular necrosis.
Bacteria, see page 593. Always culture urine prior to starting antibiotic therapy for sensitivities. Stix testing for blood or protein is of no value in the diagnosis of a UTI as both can be absent in the urine of many people with bacteriuria.
Blood and quantitative tests
The use of serum urea, creatinine and GFR as measures of renal function is discussed on page 564. Other quantitative tests of disturbed renal function are described under the relevant disorders, as are diagnostic tests, e.g. ANCA, immunofluorescence and complement.
Imaging techniques
Plain X-ray
A plain radiograph of the abdomen is valuable to identify renal calcification or radiodense calculi in the kidney, renal pelvis, line of the ureters or bladder (Fig. 12.7).
Ultrasonography
Renal measurement and for renal biopsy or other interventional procedures
Checking for pelvicalyceal dilatation as an indication of renal obstruction when chronic renal obstruction is suspected. (In suspected acute ureteric obstruction, unenhanced spiral CT is the method of choice.)
Characterizing renal masses as cystic or solid
Diagnosing polycystic kidney disease
Detecting intrarenal and/or perinephric fluid (e.g. pus, blood)
Demonstrating renal arterial perfusion or detecting renal vein thrombosis using Doppler. Doppler ultrasonography (duplex) has the advantage of being non-invasive and is based on the principle that, when incident sound waves are reflected from a moving structure, their frequency is shifted by an amount proportional to the velocity of the reflector (e.g. an RBC); this shift can be quantified and displayed as a spectral Doppler scan or colour overlay (colour Doppler). However, duplex imaging is limited by central obesity, bowel gas and certain body habitus characteristics. Moreover, it is technically demanding, highly operator dependent, and is not universally available. It is at best a screening initial investigation and always requires confirmation by more reliable imaging techniques (CTA/MRA see below) if renal stenosis is suspected
Measurement of bladder wall thickness in a distended bladder and to check for bladder tumours and stones. A scan obtained after voiding allows bladder emptying to be assessed.
The disadvantages of using ultrasonography to assess the urinary tract are:
It does not show detailed pelvicalyceal anatomy
It does not fully visualize the normal adult ureter
It may miss small renal calculi and does not detect the majority of ureteric calculi
Computed tomography (CT)
Characterize renal masses which are indeterminate at ultrasonography
Detect ‘lucent’ calculi (low-density calculi which are lucent on plain films, e.g. uric acid stones, are well seen on CT)
Evaluate the retroperitoneum for tumours, retroperitoneal fibrosis (periaortitis) and other causes of ureteric obstruction
Visualize the renal arteries and veins by CT angiography
Stage bladder and prostate tumours (MRI is increasingly used instead to stage prostate cancer).
Disadvantages include radiation and contrast nephrotoxicity (p. 614).
Magnetic resonance imaging (MRI)
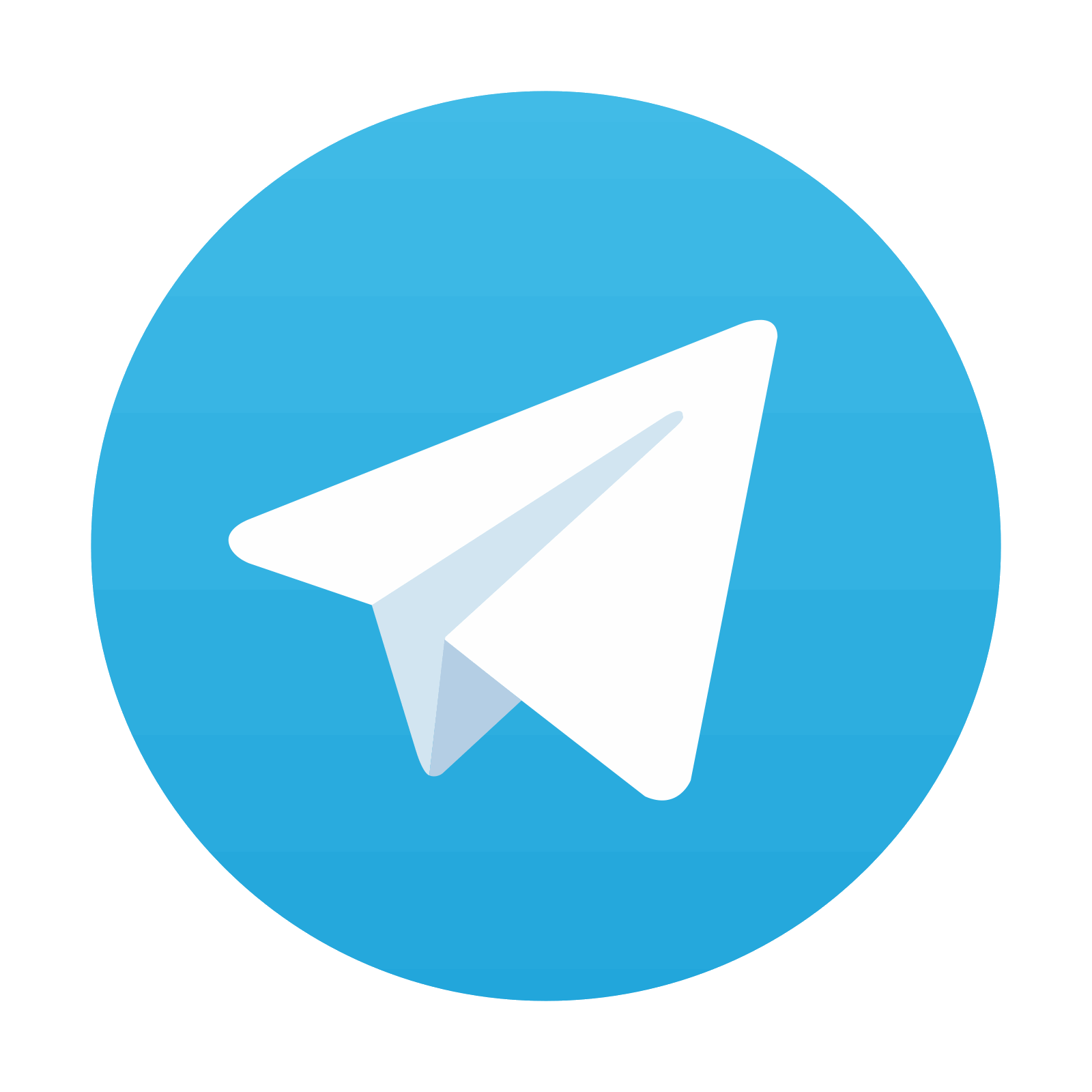
Stay updated, free articles. Join our Telegram channel
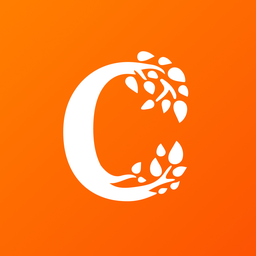
Full access? Get Clinical Tree
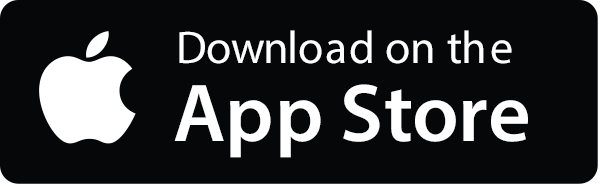
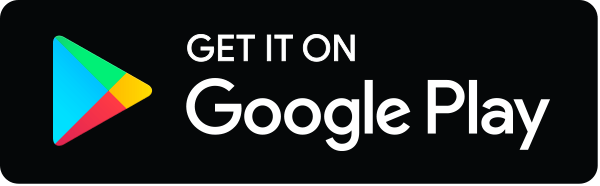
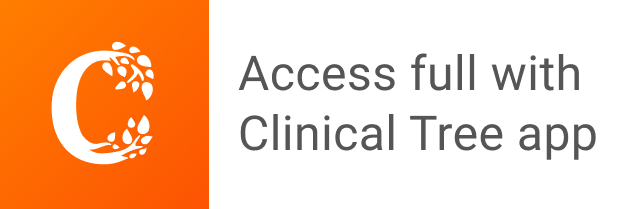