During fasting, many of the reactions of glycolysis are reversed as the liver produces glucose to maintain blood glucose levels. This process of glucose production is called gluconeogenesis.
Gluconeogenesis, which occurs primarily in the liver, is the pathway for the synthesis of glucose from compounds other than carbohydrates. In humans, the major precursors of glucose are lactate, glycerol, and amino acids, particularly alanine. Except for three key sequences, the reactions of gluconeogenesis are reversals of the steps of glycolysis (Fig. 28.1). The sequences of gluconeogenesis that do not use enzymes of glycolysis involve the irreversible, regulated steps of glycolysis. These three sequences are the conversion of (1) pyruvate to phosphoenolpyruvate (PEP), (2) fructose 1,6-bisphosphate to fructose 6-phosphate (fructose 6-P), and (3) glucose 6-phosphate (glucose 6-P) to glucose.
FIGURE 28.1 Glycolysis and gluconeogenesis in the liver. The gluconeogenic pathway is almost the reverse of the glycolytic pathway, except for three reaction sequences. At these three steps, the reactions are catalyzed by different enzymes. The energy requirements of these reactions differ, and one pathway can be activated while the other is inhibited. The steps for which enzyme names are indicated are the irreversible steps of those pathways. All other steps are reversible, although for clarity this is not indicated in the figure. Acetyl CoA, acetyl coenzyme A; ADP, adenosine diphosphate; ATP, adenosine triphosphate; DHAP, dihydroxyacetone phosphate; fructose-1,6-P, fructose 1,6-bisphosphate; fructose-6-P, fructose 6-phosphate; GDP, guanosine diphosphate; glucose-6-P, glucose 6-phosphate; glyceraldehyde-3-P, glyceraldehyde 3-phosphate; glycerol-3-P, glycerol 3-phosphate; GTP, guanosine triphosphate; NAD, nicotinamide adenine dinucleotide; OAA, oxaloacetate; PEP, phosphoenolpyruvate; Pi, inorganic phosphate; TCA, tricarboxylic acid.
Some tissues of the body, such as the brain and red blood cells, cannot synthesize glucose on their own but depend on glucose for energy. On a long-term basis, most tissues also require glucose for other functions, such as synthesis of the ribose moiety of nucleotides or the carbohydrate portion of glycoproteins and glycolipids. Therefore, to survive, humans must have mechanisms for maintaining blood glucose levels.
After a meal containing carbohydrates, blood glucose levels rise (Fig. 28.2). Some of the glucose from the diet is stored in the liver as glycogen. After 2 or 3 hours of fasting, this glycogen begins to be degraded by the process of glycogenolysis, and glucose is released into the blood. As glycogen stores decrease, adipose triacylglycerols are also degraded, providing fatty acids as an alternative fuel and glycerol for the synthesis of glucose by gluconeogenesis. Amino acids are also released from the muscle to serve as gluconeogenic precursors.
FIGURE 28.2 Sources of blood glucose in the fed, fasting, and starved states. RBC, red blood cells.
During an overnight fast, blood glucose levels are maintained by both glycogenolysis and gluconeogenesis. However, after approximately 30 hours of fasting, liver glycogen stores are mostly depleted. Subsequently, gluconeogenesis is the only source of blood glucose.
Changes in the metabolism of glucose that occur during the switch from the fed to the fasting state are regulated by the hormones insulin and glucagon. Insulin is elevated in the fed state, and glucagon is elevated during fasting. Insulin stimulates the transport of glucose into certain cells such as those in muscle and adipose tissue. Insulin also alters the activity of key enzymes that regulate metabolism, stimulating the storage of fuels. Glucagon counters the effects of insulin, stimulating the release of stored fuels and the conversion of lactate, amino acids, and glycerol to glucose.
Blood glucose levels are maintained not only during fasting but also during exercise, when muscle cells take up glucose from the blood and oxidize it for energy. During exercise, the liver supplies glucose to the blood by the processes of glycogenolysis and gluconeogenesis.
THE WAITING ROOM 
Al M., who suffers from alcohol use disorder, was brought to the emergency department by his landlady, who stated that he had been drinking heavily for the past week. During this time, his appetite had gradually diminished, and he had not eaten any food for the past 3 days. He was confused, combative, tremulous, and sweating profusely. His speech was slurred. His heart rate was rapid (110 beats/minute). As his blood pressure was being determined, he had a grand mal seizure. His blood glucose, drawn just before the onset of the seizure, was 28 mg/dL or 1.6 mM (reference range for overnight fasting blood glucose, 70 to 100 mg/dL or 3.9 to 5.6 mM). His blood ethanol level drawn at the same time was 295 mg/dL (intoxication level, i.e., “confused” stage, 150 to 300 mg/dL).
Emma W. presented to the emergency department 3 days after being discharged from the hospital following a 7-day admission for a severe asthma exacerbation. During her last admission, she was intubated and required high-dose intravenous methylprednisolone (a synthetic anti-inflammatory glucocorticoid) for the first 4 days of her stay. After 3 additional days on oral prednisone, she was discharged on substantial pharmacologic doses of this steroid and instructed to return to her physician’s office in 5 days. She presented now with marked polyuria (increased urination), polydipsia (increased thirst), and muscle weakness. Her blood glucose was 275 mg/dL or 15 mM (reference range, 70 to 100 mg/dL or 3.9 to 5.6 mM).
Dianne A. took her morning insulin, but then did not feel well, so did not eat and took a nap. When her friend came over later, she was unresponsive. The friend called an ambulance, and Dianne A. was rushed to the hospital emergency department in a coma. Her pulse and blood pressure at admission were normal. Her skin was flushed and slightly moist. Her respirations were slightly slow.
Ann R. continues to resist efforts on the part of her psychiatrist and family physician to convince her to increase her caloric intake. Her body weight varies between 97 and 99 lb, far below the desirable weight for a woman who is 5 ft 7 in tall. In spite of her severe diet, her fasting blood glucose levels range from 55 to 70 mg/dL. She denies having any hypoglycemic symptoms.
Otto S. has complied with his calorie-restricted diet and aerobic exercise program. He has lost another 7 lb and is closing in on his goal of weighing 154 lb. He notes increasing energy during the day, and he remains alert during lectures and assimilates the lecture material noticeably better than he did before starting his weight loss and exercise program. He jogs for 45 minutes each morning before breakfast.
I. Glucose Metabolism in the Liver
Glucose serves as a fuel for most tissues of the body. It is the major fuel for certain tissues such as the brain and red blood cells. After a meal, food is the source of blood glucose. The liver oxidizes glucose and stores the excess as glycogen. The liver also uses the pathway of glycolysis to convert glucose to pyruvate, which provides carbon for the synthesis of fatty acids. Glycerol 3-phosphate, produced from glycolytic intermediates, combines with fatty acids to form triacylglycerols, which are secreted into the blood in very-low-density lipoprotein (VLDL; explained further in Chapter 31). During fasting, the liver releases glucose into the blood, so that glucose-dependent tissues do not suffer from a lack of energy. Two mechanisms are involved in this process: glycogenolysis and gluconeogenesis. Hormones, particularly insulin and glucagon, dictate whether glucose flows through glycolysis or whether the reactions are reversed and glucose is produced via gluconeogenesis.
II. Gluconeogenesis
Gluconeogenesis, the process by which glucose is synthesized from noncarbohydrate precursors, occurs mainly in the liver under fasting conditions. Under the more extreme conditions of starvation, the kidney cortex also may produce glucose. For the most part, the glucose produced by the kidney cortex is used by the kidney medulla, but some may enter the bloodstream.
Starting with pyruvate, most of the steps of gluconeogenesis are simply reversals of those of glycolysis (Fig. 28.3). In fact, these pathways differ at only three points. Enzymes involved in catalyzing these steps are regulated so that either glycolysis or gluconeogenesis predominates, depending on physiologic conditions.
FIGURE 28.3 Key reactions of gluconeogenesis. The precursors are amino acids (particularly alanine), lactate, and glycerol. Heavy red arrows indicate steps that differ from those of glycolysis. Dihydroxyacetone-P, dihydroxyacetone phosphate; glyceraldehyde-3-P, glyceraldehyde 3-phosphate; glycerol-3-P, glycerol 3-phosphate; Pi, inorganic phosphate; TCA, tricarboxylic acid.
Most of the steps of gluconeogenesis use the same enzymes that catalyze the process of glycolysis. The flow of carbon, of course, is in the reverse direction. Three reaction sequences of gluconeogenesis differ from the corresponding steps of glycolysis. They involve the conversion of pyruvate to phosphoenolpyruvate (PEP) and the reactions that remove phosphate from fructose 1,6-bisphosphate to form fructose 6-phosphate (fructose 6-P) and from glucose 6-phosphate (glucose 6-P) to form glucose (see Fig. 28.3). The conversion of pyruvate to PEP is catalyzed during gluconeogenesis by a series of enzymes instead of the single enzyme used for glycolysis. The reactions that remove phosphate from fructose 1,6-bisphosphate and from glucose 6-P each use single enzymes that differ from the corresponding enzymes of glycolysis. Although phosphate is added during glycolysis by kinases, which use adenosine triphosphate (ATP), it is removed during gluconeogenesis by phosphatases that release inorganic phosphate (Pi) via hydrolysis reactions.
A. Precursors for Gluconeogenesis
The three major carbon sources for gluconeogenesis in humans are lactate, glycerol, and amino acids, particularly alanine. Lactate is produced by anaerobic glycolysis in tissues such as exercising muscle or red blood cells, as well as by adipocytes during the fed state. Glycerol is released from adipose stores of triacylglycerol, and amino acids come mainly from amino acid pools in muscle, where they may be obtained by degradation of muscle protein. Alanine, the major gluconeogenic amino acid, is produced in the muscle from other amino acids and from glucose (see Chapter 37). Because ethanol metabolism only gives rise to acetyl coenzyme A (acetyl CoA), the carbons of ethanol cannot be used for gluconeogenesis.
B. Formation of Gluconeogenic Intermediates from Carbon Sources
The carbon sources for gluconeogenesis form pyruvate, intermediates of the tricarboxylic acid (TCA) cycle, or intermediates that are common to both glycolysis and gluconeogenesis.
1. Lactate, Amino Acids, and Glycerol
Pyruvate is produced in the liver from the gluconeogenic precursors lactate and alanine. Lactate dehydrogenase oxidizes lactate to pyruvate, generating reduced nicotinamide adenine dinucleotide (NADH; Fig. 28.4A), and alanine aminotransferase converts alanine to pyruvate (see Fig. 28.4B).
FIGURE 28.4 Metabolism of gluconeogenic precursors. A. Conversion of lactate to pyruvate. B. Conversion of alanine to pyruvate. In this reaction, alanine aminotransferase transfers the amino group of alanine to α-ketoglutarate to form glutamate. The coenzyme for this reaction, pyridoxal phosphate, accepts and donates the amino group. C. Conversion of glycerol to DHAP. α-kg, α-ketoglutarate; DHAP, dihydroxyacetone phosphate; NAD, nicotinamide adenine dinucleotide.
Although alanine is the major gluconeogenic amino acid, other amino acids, such as serine, serve as carbon sources for the synthesis of glucose because they also form pyruvate, the substrate for the initial step in the process. Some amino acids form intermediates of the TCA cycle (see Chapter 23), which can enter the gluconeogenic pathway.
The carbons of glycerol are gluconeogenic because they form dihydroxyacetone phosphate (DHAP), a glycolytic intermediate (see Fig. 28.4C).
2. Propionate
Fatty acids with an odd number of carbon atoms, which are obtained mainly from vegetables in the diet, produce propionyl coenzyme A (propionyl CoA) from the three carbons at the ω-end of the chain (see Chapter 30). These carbons are relatively minor precursors of glucose in humans. Propionyl CoA is converted to methylmalonyl coenzyme A (methylmalonyl CoA), which is rearranged to form succinyl coenzyme A (succinyl CoA), a four-carbon intermediate of the TCA cycle that can be used for gluconeogenesis. The remaining carbons of an odd-chain fatty acid form acetyl CoA, from which no net synthesis of glucose occurs. In some species, propionate is a major source of carbon for gluconeogenesis. Ruminants can produce massive amounts of glucose from propionate. In cows, the cellulose in grass is converted to propionate by bacteria in the rumen. This substrate is then used to generate >5 lb of glucose each day by the process of gluconeogenesis. Propionate can also be formed from amino acid degradation in tissues (see Chapter 37).
β-Oxidation of fatty acids produces acetyl CoA (see Chapter 30). Because the pyruvate dehydrogenase reaction is thermodynamically and kinetically irreversible, acetyl CoA does not form pyruvate for gluconeogenesis. Therefore, if acetyl CoA is to produce glucose, it must enter the TCA cycle and be converted to malate. For every two carbons of acetyl CoA that are converted to malate, two carbons are released as CO2: one in the reaction catalyzed by isocitrate dehydrogenase and the other in the reaction catalyzed by α-ketoglutarate dehydrogenase. Therefore, there is no net synthesis of glucose from acetyl CoA.
C. Pathway of Gluconeogenesis
Gluconeogenesis occurs by a pathway that reverses many, but not all, of the steps of glycolysis.
1. Conversion of Pyruvate to PEP
In glycolysis, PEP is converted to pyruvate by pyruvate kinase. In gluconeogenesis, a series of steps is required to accomplish the reversal of this reaction (Fig. 28.5). Pyruvate is carboxylated by pyruvate carboxylase to form oxaloacetate (OAA). This enzyme, which requires biotin, is the catalyst of an anaplerotic (refilling) reaction of the TCA cycle (see Chapter 23). In gluconeogenesis, this reaction replenishes the OAA that is used for the synthesis of glucose (Fig. 28.6).
FIGURE 28.5 Conversion of pyruvate to PEP in the liver. Follow the shaded circled numbers on the diagram, starting with the precursors alanine and lactate. The first step is the conversion of alanine and lactate to pyruvate. Pyruvate then enters the mitochondria and is converted to OAA (circle 2) by pyruvate carboxylase. Pyruvate dehydrogenase has been inactivated by both the NADH and acetyl CoA generated from fatty acid oxidation, which allows OAA production for gluconeogenesis. The OAA formed in the mitochondria is converted to either malate or aspartate to enter the cytoplasm via the malate/aspartate shuttle. In the cytoplasm, the malate or aspartate is converted back into OAA (circle 3), and phosphoenolpyruvate carboxykinase converts it to PEP (circle 4). The PEP formed is not converted to pyruvate, because pyruvate kinase has been inactivated by phosphorylation by the cAMP-dependent protein kinase under these conditions. The white circled numbers are alternative routes for exit of carbon from the mitochondrion using the malate/aspartate shuttle. Acetyl CoA, acetyl coenzyme A; ADP, adenosine diphosphate; ATP, adenosine triphosphate; FA, fatty acid; GDP, guanosine diphosphate; GTP, guanosine triphosphate; OAA, oxaloacetate; PEP, phosphoenolpyruvate; Pi, inorganic phosphate; PK, pyruvate kinase; TG, triacylglycerol
FIGURE 28.6 Conversion of pyruvate to oxaloacetate. ADP, adenosine diphosphate; ATP, adenosine triphosphate; Pi, inorganic phosphate.
The CO2 that was added to pyruvate to form OAA is released in the reaction catalyzed by phosphoenolpyruvate carboxykinase (PEPCK), which generates PEP (Fig. 28.7A). For this reaction, guanosine triphosphate (GTP) provides a source of energy as well as the phosphate group of PEP. Pyruvate carboxylase is found in mitochondria. In various species, PEPCK is located either in the cytosol or in mitochondria, or it is distributed between these two compartments. In humans, the enzyme is distributed about equally in each compartment.
FIGURE 28.7 Generation of PEP from gluconeogenic precursors. A. Conversion of OAA to PEP, using PEPCK. B. Interconversion of OAA and malate. C. Transamination of aspartate to form OAA. Note that the cytosolic reaction is the reverse of the mitochondrial reaction as shown in Figure 28.5. GDP, guanosine diphosphate; GTP, guanosine triphosphate; NAD, nicotinamide adenine dinucleotide; OAA, oxaloacetate; PEP, phosphoenolpyruvate; PEPCK, phosphoenolpyruvate carboxykinase.
OAA, generated from pyruvate by pyruvate carboxylase or from amino acids that form intermediates of the TCA cycle, does not readily cross the mitochondrial membrane. It is either decarboxylated to form PEP by the mitochondrial PEPCK or it is converted to malate or aspartate (see Fig. 28.7B and C). The conversion of OAA to malate requires NADH. PEP, malate, and aspartate can be transported into the cytosol.
After malate or aspartate traverses the mitochondrial membrane (acting as a carrier of OAA) and enters the cytosol, it is reconverted to OAA by reversal of the reactions given previously (see Fig. 28.7B and C). The conversion of malate to OAA generates NADH. Whether OAA is transported across the mitochondrial membrane as malate or aspartate depends on the need for reducing equivalents in the cytosol. NADH is required to reduce 1,3-bisphosphoglycerate to glyceraldehyde 3-phosphate during gluconeogenesis.
OAA, produced from malate or aspartate in the cytosol, is converted to PEP by the cytosolic PEPCK (see Fig. 28.7A).
2. Conversion of PEP to Fructose 1,6-Bisphosphate
The remaining steps of gluconeogenesis occur in the cytosol (Fig. 28.8). Starting with PEP as a substrate, the steps of glycolysis are reversed to form glyceraldehyde 3-phosphate. For every two molecules of glyceraldehyde 3-phosphate that are formed, one is converted to DHAP. These two triose phosphates, DHAP and glyceraldehyde 3-phosphate, condense to form fructose 1,6-bisphosphate by a reversal of the aldolase reaction. Because glycerol forms DHAP, it enters the gluconeogenic pathway at this level.
FIGURE 28.8 Conversion of phosphoenolpyruvate and glycerol to glucose. Gluconeogenic reactions are indicated by the red arrows. Glucokinase is inactive because of the low levels of glucose in the cell (below the Km for glucokinase), whereas PFK-1 is inactive because of the low concentration of the allosteric activators AMP and fructose 2,6-bisphosphate, coupled to high concentrations of ATP, an allosteric inhibitor. ADP, adenosine diphosphate; AMP, adenosine monophosphate; ATP, adenosine triphosphate; F-2,6-P, fructose 2,6-bisphosphate; NAD, nicotinamide adenine dinucleotide; PFK-1, phosphofructokinase-1; Pi, inorganic phosphate.
3. Conversion of Fructose 1,6-Bisphosphate to Fructose 6-P
The enzyme fructose 1,6-bisphosphatase releases inorganic phosphate from fructose 1,6-bisphosphate to form fructose 6-P. This is not a reversal of the phosphofructokinase-1 (PFK-1) reaction; ATP is not produced when the phosphate is removed from the 1-position of fructose 1,6-bisphosphate because that is a low-energy phosphate bond. Rather, inorganic phosphate is released in this hydrolysis reaction. In the next reaction of gluconeogenesis, fructose 6-P is converted to glucose 6-P by the same isomerase used in glycolysis (phosphoglucoisomerase).
4. Conversion of Glucose 6-P to Glucose
Glucose 6-phosphatase hydrolyzes Pi from glucose 6-P, and free glucose is released into the blood. As with fructose 1,6-bisphosphatase, this is not a reversal of the glucokinase reaction, because the phosphate bond in glucose 6-P is a low-energy bond, and ATP is not generated at this step.
Glucose 6-phosphatase is located in the membrane of the endoplasmic reticulum. It is used not only in gluconeogenesis, but also to produce blood glucose from the breakdown of liver glycogen.
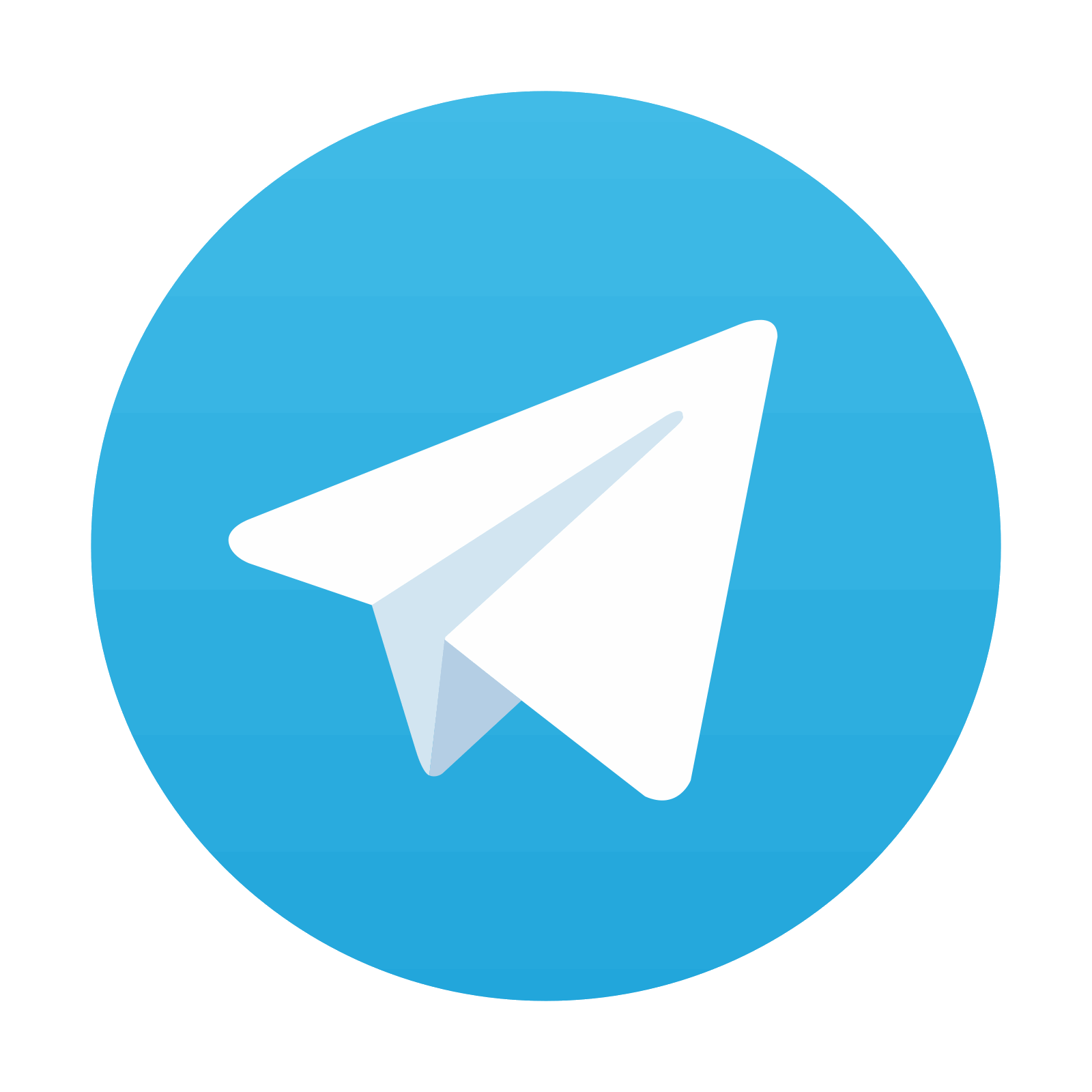
Stay updated, free articles. Join our Telegram channel
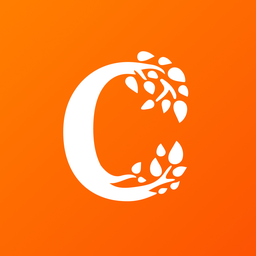
Full access? Get Clinical Tree
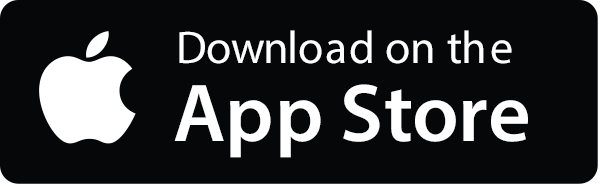
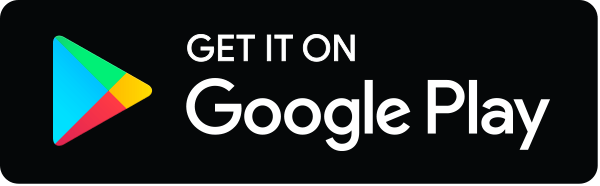