25
General Anesthetics
CASE STUDY
An elderly man with type 2 diabetes mellitus and ischemic pain in the lower extremity is scheduled for femoral-to-popliteal bypass surgery. He has a history of hypertension and coronary artery disease with symptoms of stable angina and can walk only half a block before pain in his legs forces him to stop. He has a 50-pack-a-year smoking history but stopped 2 years ago. His medications include atenolol, atorvastatin, and hydrochlorothiazide. The nurse in the preoperative holding area obtains the following vital signs: temperature 36.8°C (98.2°F), blood pressure 168/100 mm Hg, heart rate 78 bpm, oxygen saturation by pulse oximeter 96% while breathing room air, pain 5/10 in the right lower leg. What anesthetic agents will you choose and why? Does the choice of anesthetic make a difference?
For centuries, humankind has relied on natural medicines and physical methods to control surgical pain. Historical texts describe the sedative effects of cannabis, henbane, mandrake, and opium poppy. Physical methods such as cold, nerve compression, carotid artery occlusion, and cerebral concussion were also employed, with variable effect. Although surgery was performed under ether anesthesia as early as 1842, the first public demonstration of surgical general anesthesia in 1846 is generally accepted as the start of the modern era of anesthesia. For the first time physicians had a reliable means to keep their patients from experiencing pain during surgical procedures.
The neurophysiologic state produced by general anesthetics is characterized by five primary effects: unconsciousness, amnesia, analgesia, inhibition of autonomic reflexes, and skeletal muscle relaxation. None of the currently available anesthetic agents when used alone can achieve all five of these desired effects well. In addition, an ideal anesthetic drug should induce rapid, smooth loss of consciousness, be rapidly reversible upon discontinuation, and possess a wide margin of safety.
The modern practice of anesthesiology relies on the use of combinations of intravenous and inhaled drugs (balanced anesthesia techniques) to take advantage of the favorable properties of each agent while minimizing their adverse effects. The choice of anesthetic technique is determined by the type of diagnostic, therapeutic, or surgical intervention to be performed. For minor superficial surgery or for invasive diagnostic procedures, oral or parenteral sedatives can be used in combination with local anesthetics, so-called monitored anesthesia care techniques (see Box: Sedation & Monitored Anesthesia Care, and Chapter 26). These techniques provide profound analgesia, with retention of the patient’s ability to maintain a patent airway and to respond to verbal commands. For more extensive surgical procedures, anesthesia may begin with preoperative benzodiazepines, be induced with an intravenous agent (eg, thiopental or propofol), and be maintained with a combination of inhaled (eg, volatile agents, nitrous oxide) or intravenous drugs (eg, propofol, opioid analgesics), or both.
MECHANISM OF GENERAL ANESTHETIC ACTION
General anesthetics have been in clinical use for more than 160 years but their mechanism of action remains unknown. Initial research focused on identifying a single biologic site of action for these drugs. In recent years this “unitary theory” of anesthetic action has been supplanted by a more complex picture of molecular targets located at multiple levels of the central nervous system (CNS).
Anesthetics affect neurons at various cellular locations, but the primary focus has been on the synapse. A presynaptic action may alter the release of neurotransmitters, whereas a postsynaptic effect may change the frequency or amplitude of impulses exiting the synapse. At the organ level, the effect of anesthetics may result from strengthening inhibition or from diminishing excitation within the CNS. Studies on isolated spinal cord tissue have demonstrated that excitatory transmission is impaired more strongly by anesthetics than inhibitory effects are potentiated.
Many diagnostic and minor therapeutic surgical procedures can be performed without general anesthesia using sedation-based anesthetic techniques. In this setting, regional or local anesthesia supplemented with midazolam or propofol and opioid analgesics (or ketamine) may be a more appropriate and safer approach than general anesthesia for superficial surgical procedures. This anesthetic technique is known as monitored anesthesia care, often abbreviated as MAC, not to be confused with the minimal alveolar concentration for the comparison of potencies of inhaled anesthetics (see text and Box: What Does Anesthesia Represent & Where Does It Work?). The technique typically involves the use of intravenous midazolam for premedication (to provide anxiolysis, amnesia, and mild sedation) followed by a titrated, variable-rate propofol infusion (to provide moderate to deep levels of sedation). A potent opioid analgesic or ketamine may be added to minimize the discomfort associated with the injection of local anesthesia and the surgical manipulations.
Another approach, used primarily by nonanesthesiologists, is called conscious sedation. This technique refers to drug-induced alleviation of anxiety and pain in combination with an altered level of consciousness associated with the use of smaller doses of sedative medications. In this state the patient retains the ability to maintain a patent airway and is responsive to verbal commands. A wide variety of intravenous anesthetic drugs have proved to be useful drugs in conscious sedation techniques (eg, diazepam, midazolam, propofol). Use of benzodiazepines and opioid analgesics (eg, fentanyl) in conscious sedation protocols has the advantage of being reversible by the specific receptor antagonist drugs (flumazenil and naloxone, respectively).
A specialized form of sedation is occasionally required in the intensive care unit (ICU), when patients are under severe stress and require mechanical ventilation for prolonged periods. In this situation, sedative-hypnotic drugs and low doses of intravenous anesthetics may be combined. Recently, dexmedetomidine has become a popular choice for this indication.
Deep sedation is similar to a light state of general anesthesia characterized by decreased consciousness from which the patient is not easily aroused. The transition from deep sedation to general anesthesia is fluid and can be difficult to define. Because deep sedation is often accompanied by a loss of protective reflexes, an inability to maintain a patent airway and lack of verbal responsiveness to surgical stimuli, this state may be indistinguishable from general anesthesia. A practitioner with expertise in airway management, such as an anesthesiologist or nurse anesthetist, must be present.
Intravenous agents used in deep sedation protocols mainly include the sedative-hypnotics propofol and midazolam, sometimes in combination with potent opioid analgesics or ketamine, depending on the level of pain associated with the surgery or procedure.
Chloride channels (γ-aminobutyric acid-A [GABAA] and glycine receptors) and potassium channels (K2P, possibly KV, and KATP channels) remain the primary inhibitory ion channels considered legitimate candidates of anesthetic action. Excitatory ion channel targets include those activated by acetylcholine (nicotinic and muscarinic receptors), by glutamate (amino-3-hydroxy-5-methyl-4-isoxazol-propionic acid [AMPA], kainate, and N-methyl-D-aspartate [NMDA] receptors), or by serotonin (5-HT2 and 5-HT3 receptors). Figure 25–1 depicts the relation of these inhibitory and excitatory targets of anesthetics within the context of the nerve terminal.
FIGURE 25–1 Putative targets of anesthetic action. Anesthetic drugs may (A) enhance inhibitory synaptic activity or (B) diminish excitatory activity. ACh, acetylcholine; GABAA, γ-aminobutyric acid-A.
INHALED ANESTHETICS
A clear distinction should be made between volatile and gaseous anesthetics, both of which are administered by inhalation. Volatile anesthetics (halothane, enflurane, isoflurane, desflurane, sevoflurane) have low vapor pressures and thus high boiling points so that they are liquids at room temperature (20°C) and sea-level ambient pressure, whereas gaseous anesthetics (nitrous oxide, xenon) have high vapor pressures and low boiling points such that they are in gas form at room temperature. The special characteristics of volatile anesthetics make it necessary that they be administered using vaporizers. Figure 25–2 shows the chemical structures of important, clinically used, inhaled anesthetics.
FIGURE 25–2 Chemical structures of inhaled anesthetics.
PHARMACOKINETICS
Inhaled anesthetics, volatile as well as gaseous, are taken up through gas exchange in the alveoli of the lung. Uptake from the alveoli into the blood and distribution and partitioning into the effect compartments are important determinants of the kinetics of these agents. As previously mentioned, an ideal anesthetic should have a rapid onset (induction), and its effect should be rapidly terminated. To achieve this, the effect site concentration in the CNS (brain and spinal cord) will need to change rapidly. Several factors determine how quickly the CNS concentration changes.
Uptake & Distribution
A. Factors Controlling Uptake
1. Inspired concentration and ventilation—The driving force for uptake of an inhaled anesthetic into the body is the alveolar concentration. Two parameters that can be controlled by the anesthesiologist determine how quickly the alveolar concentration changes: (1) inspired concentration or partial pressure, and (2) alveolar ventilation. The partial pressure of an inhaled anesthetic in the inspired gas mixture directly affects the maximum partial pressure that can be achieved in the alveoli as well as the rate of increase of the partial pressure in the alveoli. Increases in the inspired partial pressure increase the gradient between inspired and alveolar partial pressure to accelerate induction. The increase of partial pressure in the alveoli is usually expressed as a ratio of alveolar concentration (FA) over inspired concentration (FI); the faster FA/FI approaches 1 (representing inspired-to-alveolar equilibrium), the faster anesthesia will occur during an inhaled induction.
The primary parameter other than inspired concentration that directly controls the rate by which FA/FI approaches 1 is alveolar ventilation. An increase in ventilation will increase the rate of rise. The magnitude of the effect varies according to the blood:gas partition coefficient. An increase in pulmonary ventilation is accompanied by only a slight increase in arterial tension of an anesthetic with low blood solubility, but can significantly increase tension of agents with moderate to high blood solubility (Figure 25–3). For example, a fourfold increase in the ventilation rate almost doubles the FA/FI ratio for halothane during the first 10 minutes of administration but increases the FA/FI ratio for nitrous oxide by only 15%. Thus, hyperventilation increases the speed of induction of anesthesia with inhaled anesthetics that would normally have a slow onset. Depression of respiration by opioid analgesics slows the onset of anesthesia of inhaled anesthetics unless ventilation is manually or mechanically assisted.
FIGURE 25–3 Effect of ventilation on FA/FI and induction of anesthesia. Increased ventilation (8 L/min versus 2 L/min) accelerates the rate of rise toward equilibration of both halothane and nitrous oxide but results in a larger percentage increase for halothane in the first few minutes of induction.
2. Solubility—As described above, the rate of rise of FA/FI is an important determinant of the speed of induction, but is opposed by the uptake of anesthetic into the blood. Uptake is determined by pharmacokinetic characteristics of each anesthetic agent as well as patient factors.
One of the most important factors influencing the transfer of an anesthetic from the lungs to the arterial blood is its solubility characteristics (Table 25–1). The blood:gas partition coefficient is a useful index of solubility and defines the relative affinity of an anesthetic for the blood compared with that of inspired gas. The partition coefficients for desflurane and nitrous oxide, which are relatively insoluble in blood, are extremely low. When an anesthetic with low blood solubility diffuses from the lung into the arterial blood, relatively few molecules are required to raise its partial pressure; therefore, the arterial tension rises rapidly (Figure 25–4, top; nitrous oxide, desflurane, sevoflurane). Conversely, for anesthetics with moderate to high solubility (Figure 25–4, bottom; halothane, isoflurane), more molecules dissolve in the blood before partial pressure changes significantly, and arterial tension of the gas increases less rapidly. A blood:gas partition coefficient of 0.47 for nitrous oxide means that at equilibrium, the concentration in blood is less than half the concentration in the alveolar space (gas). A larger blood:gas partition coefficient produces a greater uptake of anesthetic and therefore increases the time required for FA/FI to approach equilibrium (Figure 25–4).
TABLE 25–1 Pharmacologic properties of inhaled anesthetics.
FIGURE 25–4 The alveolar anesthetic concentration (FA) approaches the inspired anesthetic concentration (FI) fastest for the least soluble agents.
3. Cardiac output—Changes in pulmonary blood flow have obvious effects on the uptake of anesthetic gases from the alveolar space. An increase in pulmonary blood flow (ie, increased cardiac output) will increase the uptake of anesthetic, thereby decreasing the rate by which FA/FI rises, which will decrease the rate of induction of anesthesia. To better understand this mechanism, one should think about the effect of cardiac output in combination with the tissue distribution and uptake of anesthetic into other tissue compartments. An increase in cardiac output and pulmonary blood flow will increase uptake of anesthetic into the blood, but the anesthetic taken up will be distributed in all tissues, not just the CNS. Cerebral blood flow is well regulated and the increased cardiac output will therefore increase delivery of anesthetic to other tissues and not the brain.
4. Alveolar-venous partial pressure difference—The anesthetic partial pressure difference between alveolar and mixed venous blood is dependent mainly on uptake of the anesthetic by the tissues, including nonneural tissues. Depending on the rate and extent of tissue uptake, venous blood returning to the lungs may contain significantly less anesthetic than arterial blood. The greater this difference in anesthetic gas tensions, the more time it will take to achieve equilibrium with brain tissue. Anesthetic uptake into tissues is influenced by factors similar to those that determine transfer of the anesthetic from the lung to the intravascular space, including tissue:blood partition coefficients, rates of blood flow to the tissues, and concentration gradients.
During the induction phase of anesthesia (and the initial phase of the maintenance period), the tissues that exert greatest influence on the arteriovenous anesthetic concentration gradient are those that are highly perfused (eg, brain, heart, liver, kidneys, and splanchnic bed). Combined, these tissues receive over 75% of the resting cardiac output. In the case of volatile anesthetics with relatively high solubility in highly perfused tissues, venous blood concentration initially is very low, and equilibrium with the alveolar space is achieved slowly.
During maintenance of anesthesia with inhaled anesthetics, the drug continues to be transferred between various tissues at rates dependent on the solubility of the agent, the concentration gradient between the blood and the tissue, and the tissue blood flow. Although muscle and skin constitute 50% of the total body mass, anesthetics accumulate more slowly in these tissues than in highly perfused tissues (eg, brain) because they receive only one fifth of the resting cardiac output. Although most anesthetic agents are highly soluble in adipose (fatty) tissues, the relatively low blood perfusion to these tissues delays accumulation, and equilibrium is unlikely to occur with most anesthetics during a typical 1- to 3-hour operation.
The combined effect of ventilation, solubility in the different tissues, cardiac output, and blood flow distribution determines the rate of rise of FA/FI characteristic of each drug. Figure 25–5 schematically compares how uptake and distribution proceeds with two widely different agents. The anesthetic state is achieved when the partial pressure of the anesthetic in the brain reaches a threshold concentration determined by its potency (MAC; see Table 25–1 and Box: What Does Anesthesia Represent & Where Does It Work?). For an insoluble agent like desflurane the alveolar partial pressure can quickly equilibrate through the blood and brain compartments to reach anesthetizing concentrations. However, for an agent like halothane, its greater solubility in blood and other tissue compartments (higher partition coefficients) produce a steeper decline in the concentration gradient from lung to brain, causing a delayed onset of anesthesia. Therefore administering a larger concentration of halothane and increasing alveolar ventilation are the two strategies that can be used by anesthesiologists to speed the rate of induction with halothane.
FIGURE 25–5 Why induction of anesthesia is slower with more soluble anesthetic gases. In this schematic diagram, solubility in blood is represented by the relative size of the blood compartment (the more soluble, the larger the compartment). Relative partial pressures of the agents in the compartments are indicated by the degree of filling of each compartment. For a given concentration or partial pressure of the two anesthetic gases in the inspired air, it will take much longer for the blood partial pressure of the more soluble gas (halothane) to rise to the same partial pressure as in the alveoli. Since the concentration of the anesthetic agent in the brain can rise no faster than the concentration in the blood, the onset of anesthesia will be slower with halothane than with nitrous oxide.
B. Elimination
Recovery from inhalation anesthesia follows some of the same principles in reverse that are important during induction. The time to recovery from inhalation anesthesia depends on the rate of elimination of the anesthetic from the brain. One of the most important factors governing rate of recovery is the blood:gas partition coefficient of the anesthetic agent. Other factors controlling rate of recovery include pulmonary blood flow, magnitude of ventilation, and tissue solubility of the anesthetic. Two features differentiate the recovery phase from the induction phase. First, transfer of an anesthetic from the lungs to blood can be enhanced by increasing its concentration in inspired air, but the reverse transfer process cannot be enhanced because the concentration in the lungs cannot be reduced below zero. Second, at the beginning of the recovery phase, the anesthetic gas tension in different tissues may be quite variable, depending on the specific agent and the duration of anesthesia. In contrast, at the start of induction of anesthesia the initial anesthetic tension is zero in all tissues.
Inhaled anesthetics that are relatively insoluble in blood (ie, possess low blood:gas partition coefficients) and brain are eliminated faster than the more soluble anesthetics. The washout of nitrous oxide, desflurane, and sevoflurane occurs at a rapid rate, leading to a more rapid recovery from their anesthetic effects compared with halothane and isoflurane. Halothane is approximately twice as soluble in brain tissue and five times more soluble in blood than nitrous oxide and desflurane; its elimination therefore takes place more slowly, and recovery from halothane- and isoflurane-based anesthesia is predictably less rapid.
The duration of exposure to the anesthetic can also have a significant effect on the recovery time, especially in the case of the more soluble anesthetics (eg, halothane and isoflurane). Accumulation of anesthetics in muscle, skin, and fat increases with prolonged exposure (especially in obese patients), and blood tension may decline slowly during recovery as the anesthetic is slowly eliminated from these tissues. Although recovery may be rapid even with the more soluble agents following a short period of exposure, recovery is slow after prolonged administration of halothane or isoflurane.
1. Ventilation—Two parameters that can be manipulated by the anesthesiologist are useful in controlling the speed of induction of and recovery from inhaled anesthesia: (1) concentration of anesthetic in the inspired gas and (2) alveolar ventilation. Because the concentration of anesthetic in the inspired gas cannot be reduced below zero, hyperventilation is the only way to speed recovery.
2. Metabolism—Modern inhaled anesthetics are eliminated mainly by ventilation and are only metabolized to a very small extent; thus, metabolism of these drugs does not play a significant role in the termination of their effect. However, metabolism may have important implications for their toxicity (see Toxicity of Anesthetic Agents). Hepatic metabolism may also contribute to the elimination of and recovery from some older volatile anesthetics. For example, halothane is eliminated more rapidly during recovery than enflurane, which would not be predicted from their respective tissue solubility. This increased elimination occurs because over 40% of inspired halothane is metabolized during an average anesthetic procedure, whereas less than 10% of enflurane is metabolized over the same period.
In terms of the extent of hepatic metabolism, the rank order for the inhaled anesthetics is halothane > enflurane > sevoflurane > isoflurane > desflurane > nitrous oxide (Table 25–1). Nitrous oxide is not metabolized by human tissues. However, bacteria in the gastrointestinal tract may be able to break down the nitrous oxide molecule.
PHARMACODYNAMICS
Organ System Effects of Inhaled Anesthetics
A. Cerebral Effects
Anesthetic potency is currently described by the minimal alveolar concentration (MAC) required to prevent a response to a surgical incision (see Box: What Does Anesthesia Represent & Where Does It Work?).
Inhaled anesthetics (and intravenous anesthetics, discussed later) decrease the metabolic activity of the brain. Decreased cerebral metabolic rate (CMR) generally reduces blood flow within the brain. However, volatile anesthetics also cause cerebral vasodilation, which can increase cerebral blood flow. The net effect on cerebral blood flow (increase, decrease, or no change) depends on the concentration of anesthetic delivered. At 0.5 MAC, the reduction in CMR is greater than the vasodilation caused by the anesthetic, so cerebral blood flow is decreased. Conversely, at 1.5 MAC, vasodilation by the anesthetic is greater than the reduction in CMR, so cerebral blood flow is increased. In between, at 1.0 MAC, the effects are balanced and cerebral blood flow is unchanged. An increase in cerebral blood flow is clinically undesirable in patients who have increased intracranial pressure because of brain tumor, intracranial hemorrhage, or head injury. Therefore, administration of high concentrations of volatile anesthetics is undesirable in patients with increased intracranial pressure. Hyperventilation can be used to attenuate this response; decreasing the PaCO2 (the partial pressure of carbon dioxide in arterial blood) through hyperventilation causes cerebral vasoconstriction. If the patient is hyperventilated before the volatile agent is started, the increase in intracranial pressure can be minimized.
Nitrous oxide can increase cerebral blood flow and cause increased intracranial pressure. This effect is most likely caused by activation of the sympathetic nervous system (as described below). Therefore, nitrous oxide may be combined with other agents (intravenous anesthetics) or techniques (hyperventilation) that reduce cerebral blood flow in patients with increased intracranial pressure.
What Does Anesthesia Represent & Where Does It Work?
Anesthetic action has three principal components: immobility, amnesia, and unconsciousness.
Immobility
Immobility is the easiest anesthetic end point to measure. Edmond Eger and colleagues introduced the concept of minimal alveolar concentration (MAC) to quantify the potency of an inhalational anesthetic. They defined 1.0 MAC as the partial pressure of an inhalational anesthetic in the alveoli of the lungs at which 50% of a population of nonrelaxed patients remained immobile at the time of a skin incision. Anesthetic immobility is mediated primarily by neural inhibition within the spinal cord but may also include inhibited nociceptive transmission to the brain.
Amnesia
The ablation of memory arises from several locations in the CNS, including the hippocampus, amygdala, prefrontal cortex, and regions of the sensory and motor cortices. Memory researchers differentiate two types of memory: (1) explicit memory, ie, specific awareness or consciousness under anesthesia; and (2) implicit memory, the unconscious acquisition of information under adequate levels of anesthesia. Their studies have found that formation of both types of memory is reliably prevented at low MAC values (0.2–0.4 MAC). Prevention of explicit memory (awareness) has spurred the development of monitors such as the bispectral index, electroencephalogram (EEG), and entropy monitor of auditory evoked potentials to help recognize inadequate planes of anesthesia.
Consciousness
The ability of anesthetic drugs to abolish consciousness requires action at anatomic locations responsible for the formation of human consciousness. Leading neuroscientists studying consciousness identify three regions in the brain involved in generating personal awareness: the cerebral cortex, the thalamus, and the reticular activating system. These regions seem to interact as a cortical system via identified pathways, producing a state in which humans are awake, aware, and perceiving.
Our current state of understanding supports the following framework: sensory stimuli conducted through the reticular formation of the brainstem into supratentorial signaling loops, connecting the thalamus with various regions of the cortex, are the foundation of consciousness. These neural pathways involved in the development of consciousness are disrupted by anesthetics.
Potent inhaled anesthetics produce a basic pattern of change to brain electrical activity as recorded by standard electroencephalography. Isoflurane, desflurane, sevoflurane, halothane, and enflurane produce initial activation of the EEG at low doses and then slowing of electrical activity up to doses of 1.0–1.5 MAC. At higher concentrations, EEG suppression increases to the point of electrical silence with isoflurane at 2.0–2.5 MAC. Isolated epileptic-like patterns may also be seen between 1.0 and 2.0 MAC, especially with sevoflurane and enflurane, but frank clinical seizure activity has been observed only with enflurane. Nitrous oxide used alone causes fast electrical oscillations emanating from the frontal cortex at doses associated with analgesia and depressed consciousness.
Traditionally, anesthetic effects on the brain produce four stages or levels of increasing depth of CNS depression (Guedel’s signs, derived from observations of the effects of inhaled diethyl ether): Stage I—analgesia: The patient initially experiences analgesia without amnesia. Later in stage I, both analgesia and amnesia are produced. Stage II—excitement: During this stage, the patient appears delirious, may vocalize but is completely amnesic. Respiration is rapid, and heart rate and blood pressure increase. Duration and severity of this light stage of anesthesia is shortened by rapidly increasing the concentration of the agent. Stage III—surgical anesthesia: This stage begins with slowing of respiration and heart rate and extends to complete cessation of spontaneous respiration (apnea). Four planes of stage III are described based on changes in ocular movements, eye reflexes, and pupil size, indicating increasing depth of anesthesia. Stage IV—medullary depression:
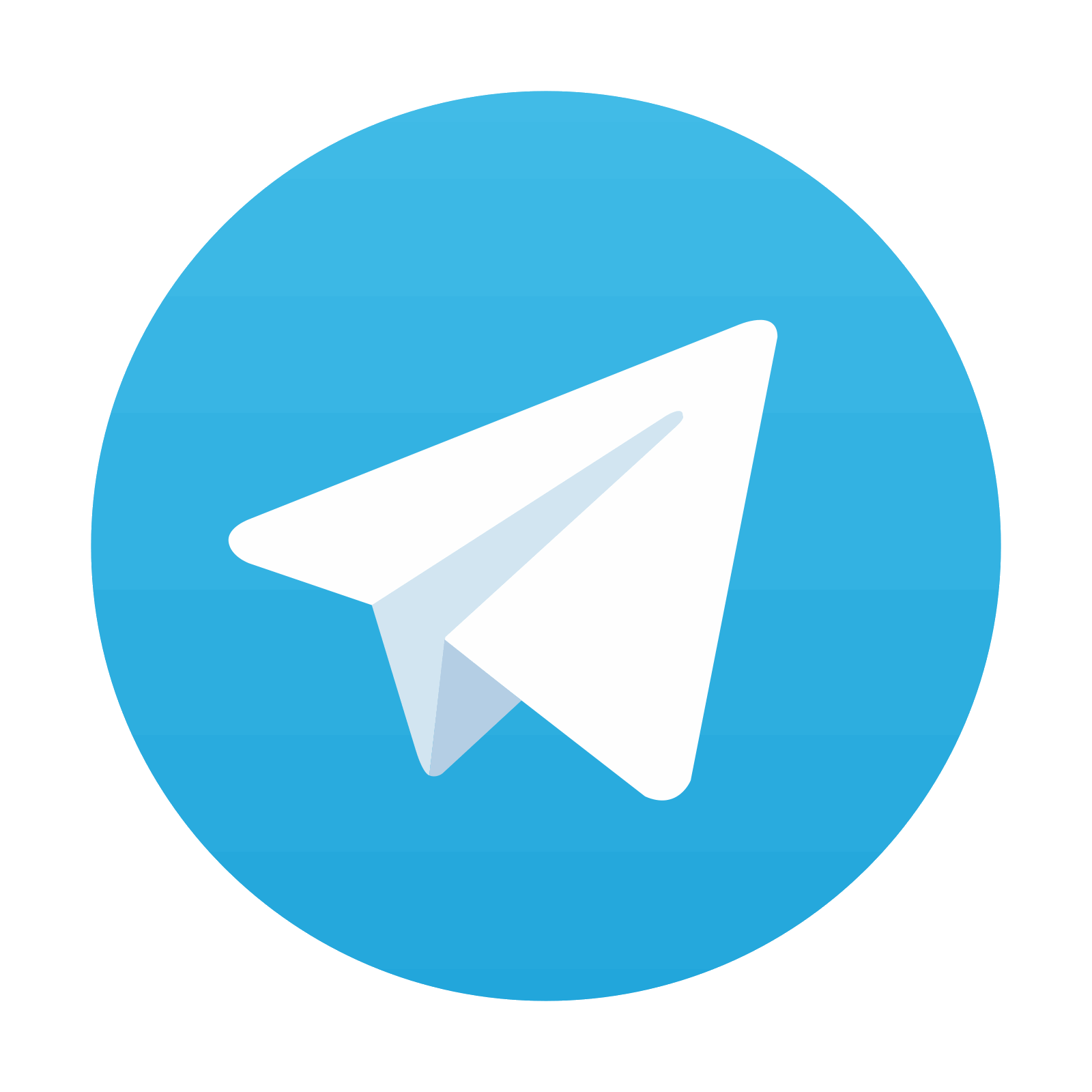
Stay updated, free articles. Join our Telegram channel
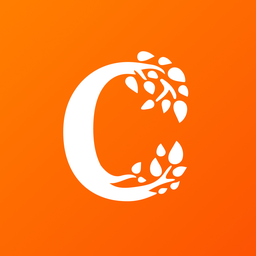
Full access? Get Clinical Tree
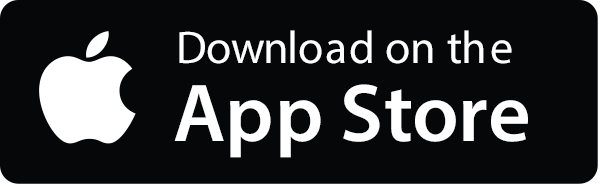
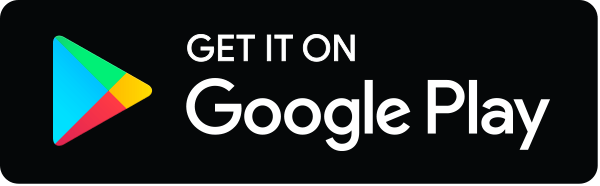