Chapter 35 General Anesthetics
Abbreviations | |
---|---|
CNS | Central nervous system |
ED50 | Median effective dose |
GABA | γ-Aminobutyric acid |
IV | Intravenous |
MAC | Minimum alveolar concentration |
N2O | Nitrous oxide |
NMDA | N-methyl-D-aspartate |
Pco2 | Carbon dioxide tension (partial pressure) |
Therapeutic Overview
The primary therapeutic considerations are summarized in the Therapeutic Overview Box.
Therapeutic Overview |
---|
Requirements of Anesthetic Drugs |
Inhalational |
Chemical stability |
Minimal irritation upon inhaling |
Speed of onset (time to loss of consciousness) |
Ability to produce analgesia, amnesia, and muscle relaxation |
Minimal side effects, especially cardiovascular and respiratory depression and toxicity to the liver |
Speed and safety of emergence |
Minimal metabolism |
Intravenous |
Chemical stability |
No pain at injection site |
Speed of onset |
Minimal side effects |
Ability to produce analgesia, amnesia, and muscle relaxation |
Speed and safety of emergence |
Rapid metabolism or redistribution |
Mechanisms of Action
The molecular basis for the anesthetic action of inhalational agents is poorly understood. Although most inhalational anesthetics contain an ether (-O-) link and a halogen (Fig. 35-1), no obvious structure-activity relationships have been defined, suggesting that they do not exert their effects through specific cell-surface receptors, unlike most other therapeutic agents acting on the central nervous system (CNS).
The potency of an inhalational anesthetic is expressed in terms of the minimum alveolar concentration (MAC), which is a concentration that prevents 50% of patients from responding to a painful stimulus, such as a skin incision. MAC is analogous to the median effective dose (ED50) and is used to express the relative potency of gaseous drugs. Meyer and Overton observed that the potencies of anesthetic agents correlate highly with their lipid solubilities, as measured by the oil:gas partition coefficient (Table 35-1; Fig. 35-2). Indeed, this relationship holds not only for agents in clinical use but also for inert gases that are not used clinically, such as xenon and argon. This correlation has given rise to several theories of anesthetic action, none of which has been substantiated.
Most IV anesthetic agents contain ring structures (Figure 35-3), have well-documented effects at specific cell-surface receptors, and include benzodiazepines, barbiturates, opioids, and several other compounds.
The barbiturate anesthetics include thiopental and methohexital, while the benzodiazepine anesthetics include diazepam and midazolam. These agents act at two distinct recognition sites on the GABAA receptor Cl− channel complex to potentiate GABA-mediated Cl− conductance and neuronal inhibition (see Chapter 31).
Several opioids used for anesthesia include fentanyl and its analogs sufentanil and remifentanil. Although morphine was used for many years, these newer high-potency compounds are gradually replacing it. The depressant action of the opioids on neuronal activity is mediated by the μ opioid receptor and those of mixed-action opioids by μ and κ opioid receptors (see Chapter 36).
Pharmacokinetics
In a mixture of gases, the partial pressure of an anesthetic agent is directly proportional to its fractional concentration in the mixture (Dalton’s Law). Thus, as depicted in Figure 35-4, in a mixture of 70% N2O, 25% O2, and 5% halothane, which might be used during mask induction of anesthesia, the partial pressures of the component gases are 532, 190, and 38 mm Hg, respectively, at 1 atmosphere (760 mm Hg) of pressure.
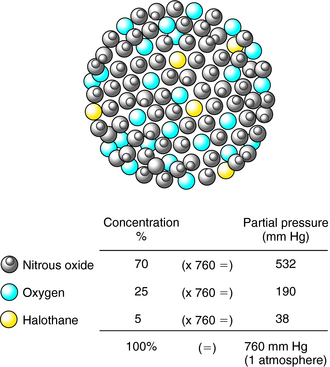
FIGURE 35–4 The partial pressure of a gas in a mixture of gases is directly proportional to its concentration.
The rate of induction of anesthesia by inhalational agents is affected by numerous factors, including those that reduce alveolar ventilation, which represents the product of the rate of respiration and tidal volume less the pulmonary dead space (Table 35-2). Thus if a patient is administered respiratory depressants such as barbiturates or opioid analgesics preoperatively, the rate of respiration or tidal volume decreases, thereby reducing alveolar ventilation in the absence of assisted ventilation. Alveolar dead space is substantial in patients with pulmonary diseases such as emphysema and atelectasis, which result in decreased alveolar ventilation and rate of anesthesia induction.
TABLE 35–2 Factors Affecting the Rate of Induction with an Inhalational Anesthetic
Condition | Rate of Induction |
---|---|
Increased concentration of anesthetic in inspired gas mixture | Increased |
Increased alveolar ventilation | Increased |
Increased solubility of anesthetic in blood (blood:gas partition coefficient) | Decreased |
Increased cardiac output | Decreased |
The path followed by an inhalational anesthetic during induction of, and emergence from, anesthesia is diagrammed in Figure 35-5. Induction is facilitated by factors that maintain a high partial pressure of the anesthetic in the inspired gas mixture, alveolar space, and arterial blood to deliver as much of the gas to the brain as quickly as possible. The alveolar membrane poses no barrier to gases, permitting unhindered diffusion in both directions. Therefore, once the anesthetic gas reaches the alveolar space, it obeys the law of mass action and moves down its partial-pressure gradient into arterial blood. At the initiation of anesthetic administration, the partial pressure of the anesthetic in the alveolar space is much higher than that in blood. Thus the partial pressure gradient between the alveolar space and the arteriolar blood is high, and initially the gas moves rapidly into blood. As the partial pressure of the anesthetic agent in blood increases, the gradient between the alveolar space and blood decreases and uptake slows (Fig. 35-6).
Another important factor in the rate of rise of the arterial partial pressure of an anesthetic gas is its solubility in blood. This relationship is expressed as the blood:gas partition coefficient. The higher the solubility is of an anesthetic gas in blood, the more must be dissolved to produce a change in partial pressure (because partial pressure is inversely proportional to solubility). This relationship is illustrated for N2O and halothane in Figure 35-7.
Nitrous oxide has a blood:gas partition coefficient of 0.47, so relatively little must be dissolved in blood for its partial pressure in blood to rise. This also is true for desflurane and sevoflurane. In contrast, blood serves as a large reservoir for halothane, retaining at equilibrium 2.3 parts for every 1 part in the alveolar space. Induction therefore depends not on dissolving the anesthetic in blood but on raising arterial partial pressure to drive the gas from blood to brain. Therefore the rate of rise of arterial partial pressure and speed of induction are fastest for gases that are least soluble in blood (see Fig. 35-6). The blood:gas partition coefficients of inhalational anesthetics are listed in Table 35-1.
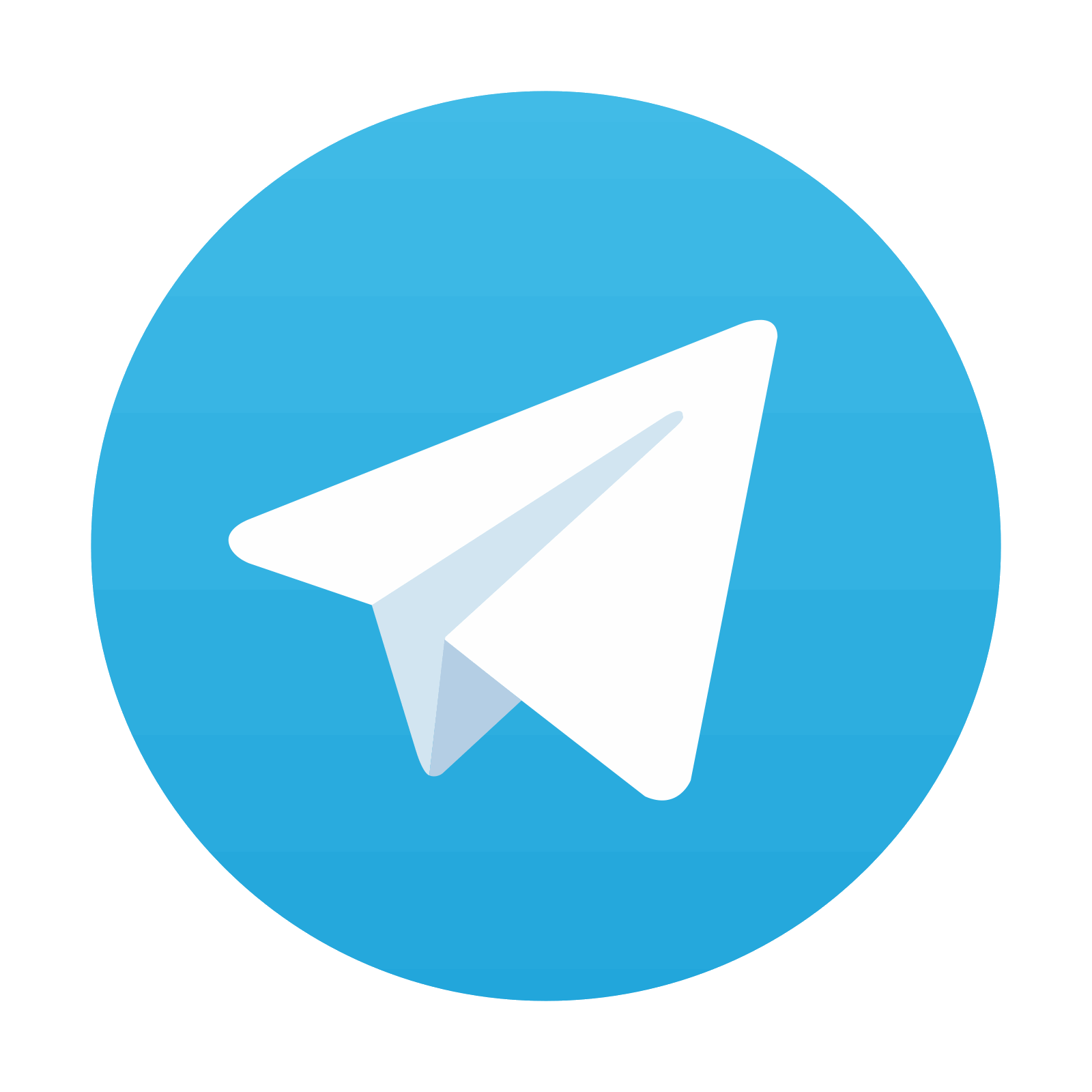
Stay updated, free articles. Join our Telegram channel
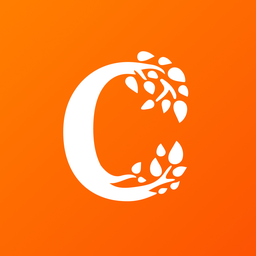
Full access? Get Clinical Tree
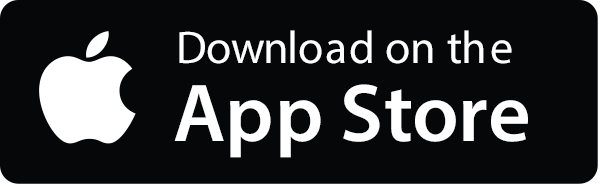
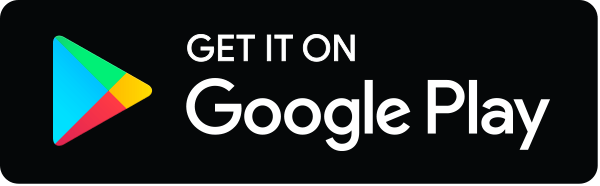
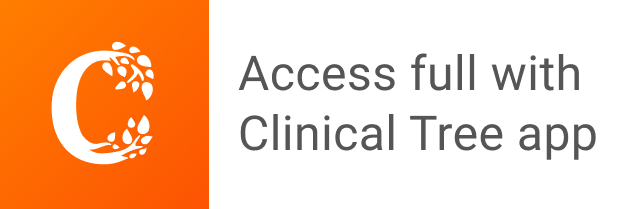