Glycogen is the storage form of glucose found in most types of cells. It is composed of glucosyl units linked by α-1,4-glycosidic bonds, with α-1,6-branches occurring roughly every 8 to 10 glucosyl units (Fig. 26.1). The liver and skeletal muscle contain the largest glycogen stores.
FIGURE 26.1 Glycogen structure. Glycogen is composed of glucosyl units linked by α-1,4-glycosidic bonds and α-1,6-glycosidic bonds. The branches occur more frequently in the center of the molecule and less frequently in the periphery. The anomeric carbon that is not attached to another glucosyl residue (the reducing end) is attached to the protein glycogenin by a glycosidic bond. The hydrogen atoms have been omitted from this figure for clarity.
The formation of glycogen from glucose is an energy-requiring pathway that begins, like most of glucose metabolism, with the phosphorylation of glucose to glucose 6-phosphate. Glycogen synthesis from glucose 6-phosphate involves the formation of uridine diphosphate glucose (UDP-glucose) and the transfer of glucosyl units from UDP-glucose to the nonreducing ends of the glycogen chains by the enzyme glycogen synthase. Once the chains reach approximately 11 glucosyl units, a branching enzyme moves 6 to 8 units to form an α-(1,6)-branch.
Glycogenolysis, the pathway for glycogen degradation, is not the reverse of the biosynthetic pathway. The degradative enzyme glycogen phosphorylase removes glucosyl units one at a time from the nonreducing ends of the glycogen chains, converting them to glucose 1-phosphate without resynthesizing UDP-glucose or UTP. A debranching enzyme removes the glucosyl residues in α-1,6 linkages.
Liver glycogen serves as a source of blood glucose. To generate glucose, the glucose 1-phosphate produced from glycogen degradation is converted to glucose 6-phosphate. Glucose 6-phosphatase, an enzyme found only in liver and kidney, converts glucose 6-phosphate to free glucose, which then enters the blood.
Glycogen synthesis and degradation are regulated in liver by hormonal changes that signal the need for blood glucose (see Chapter 19). The body maintains fasting blood glucose levels at approximately 80 mg/dL to ensure that the brain and other tissues that are dependent on glucose for the generation of adenosine triphosphate (ATP) have a continuous supply. The lack of dietary glucose, signaled by a decrease of the insulin/glucagon ratio, activates liver glycogenolysis and inhibits glycogen synthesis. Epinephrine, which signals an increased utilization of blood glucose and other fuels for exercise or emergency situations, also activates liver glycogenolysis. The hormones that regulate liver glycogen metabolism work principally through changes in the phosphorylation state of glycogen synthase in the biosynthetic pathway and glycogen phosphorylase in the degradative pathway.
In skeletal muscle, glycogen supplies glucose 6-phosphate for ATP synthesis in the glycolytic pathway. Muscle glycogen phosphorylase is stimulated during exercise by the increase of adenosine monophosphate (AMP), an allosteric activator of the enzyme, and also by phosphorylation. The phosphorylation is stimulated by calcium released during contraction, and by epinephrine, the fight-or-flight hormone. Glycogen synthesis is activated in resting muscles by the elevation of insulin after carbohydrate ingestion.
The neonate must rapidly adapt to an intermittent fuel supply after birth. Once the umbilical cord is clamped, the supply of glucose from the maternal circulation is interrupted. The combined effect of epinephrine and glucagon on the liver glycogen stores of the neonate rapidly restores glucose levels to normal.
THE WAITING ROOM 
A newborn baby girl, Gretchen C., was born after a 38-week gestation. Her 36-year-old mother developed a significant viral infection that resulted in a prolonged severe loss of appetite with nausea in the month preceding delivery, leading to minimal food intake. Fetal bradycardia (slower than normal fetal heart rate) was detected at the end of each uterine contraction of labor, a sign of possible fetal distress, and the baby was delivered emergently.
At birth, Gretchen C. was cyanotic (a bluish discoloration caused by a lack of adequate oxygenation of tissues) and limp. She responded to several minutes of assisted ventilation. Her Apgar score of 3 was low at 1 minute after birth but improved to a score of 7 at 5 minutes. The Apgar score is an objective estimate of the overall condition of the newborn, determined at both 1 and 5 minutes after birth. A score of 7, 8, or 9 is normal and fine. The highest score of 10 is less common.
Physical examination in the nursery at 10 minutes showed a thin, malnourished female newborn. Her body temperature was slightly low, her heart rate was rapid, and her respiratory rate of 55 breaths/minute was elevated. Gretchen C.’s birth weight was only 2,100 g, compared with a normal value of above 2,500 g. Her length was 47 cm, and her head circumference was 33 cm (low normal). The laboratory reported that Gretchen C.’s serum glucose level when she was unresponsive was 14 mg/dL. A glucose value below 40 mg/dL (2.5 mM) is considered to be abnormal in newborn infants.
At 5 hours of age, she was apneic (not breathing) and unresponsive. Ventilatory resuscitation was initiated, and a cannula was placed in the umbilical vein. Blood for a glucose level was drawn through this cannula, and 5 mL of a 20% glucose solution was injected. Gretchen C. slowly responded to this therapy.
Jim B., a 19-year-old bodybuilder, was rushed to the hospital emergency room in a coma. One-half hour earlier, his mother had heard a loud crashing sound in the basement where Jim B. had been lifting weights and completing his daily workout on the treadmill. She found her son on the floor having severe jerking movements of all muscles (a grand mal seizure).
On initial physical examination, he was comatose with occasional involuntary jerking movements of his extremities. Foamy saliva dripped from his mouth. He had bitten his tongue and had lost bowel and bladder control at the height of the seizure.
The laboratory reported a serum glucose level of 18 mg/dL (extremely low). The intravenous infusion of 5% glucose (5 g of glucose per 100 mL of solution), which had been started earlier, was increased to 10%. In addition, 50 g glucose was given intravenously.
I. Structure of Glycogen
Glycogen, the storage form of glucose, is a branched glucose polysaccharide composed of chains of glucosyl units linked by α-1,4-bonds with α-1,6-branches every 8 to 10 residues (see Fig. 26.1). In a molecule of this highly branched structure, only one glucosyl residue has an anomeric carbon that is not linked to another glucose residue. This anomeric carbon at the beginning of the chain is attached to the protein glycogenin. The other ends of the chains are called nonreducing ends (see Chapter 5). The branched structure permits rapid degradation and rapid synthesis of glycogen because enzymes can work on several chains simultaneously from the multiple nonreducing ends.
Glycogen is present in tissues as polymers of very high molecular weight (107 to 108 Da) collected together in glycogen particles. The enzymes involved in glycogen synthesis and degradation and some of the regulatory enzymes are bound to the surface of the glycogen particles.
II. Function of Glycogen in Skeletal Muscle and Liver
Glycogen is found in most cell types, where it serves as a reservoir of glucosyl units for adenosine triphosphate (ATP) generation from glycolysis.
Glycogen is degraded mainly to glucose 1-phosphate, which is converted to glucose 6-phosphate. In skeletal muscle and other cell types, glucose 6-phosphate enters the glycolytic pathway (Fig. 26.2). Glycogen is an extremely important fuel source for skeletal muscle when ATP demands are high and when glucose 6-phosphate is used rapidly in anaerobic glycolysis. In many other cell types, the small glycogen reservoir serves a similar purpose; it is an emergency fuel source that supplies glucose for the generation of ATP in the absence of oxygen or during restricted blood flow. In general, glycogenolysis and glycolysis are activated together in these cells.
FIGURE 26.2 Glycogenolysis in skeletal muscle and liver. Glycogen stores serve different functions in muscle cells and liver. In the muscle and most other cell types, glycogen stores serve as a fuel source for the generation of adenosine triphosphate (ATP). In the liver, glycogen stores serve as a source of blood glucose.
Glycogen serves a very different purpose in liver than in skeletal muscle and other tissues (see Fig. 26.2). Liver glycogen is the first and immediate source of glucose for the maintenance of blood glucose levels. In the liver, the glucose 6-phosphate that is generated from glycogen degradation is hydrolyzed to glucose by glucose 6-phosphatase, an enzyme that is present only in the liver and kidneys. Glycogen degradation thus provides a readily mobilized source of blood glucose as dietary glucose decreases or as exercise increases the utilization of blood glucose by muscles.
The pathways of glycogenolysis and gluconeogenesis in the liver both supply blood glucose, and, consequently, these two pathways are activated together by glucagon. Gluconeogenesis, the synthesis of glucose from amino acids and other gluconeogenic precursors (discussed in detail in Chapter 28), also forms glucose 6-phosphate, so that glucose 6-phosphatase serves as a “gateway” to the blood for both pathways (see Fig. 26.2).
III. Synthesis and Degradation of Glycogen
Glycogen synthesis, like almost all the pathways of glucose metabolism, begins with the phosphorylation of glucose to glucose 6-phosphate by hexokinase or, in the liver, glucokinase (Fig. 26.3). Glucose 6-phosphate is the precursor of glycolysis, the pentose phosphate pathway, and pathways for the synthesis of other sugars. In the pathway for glycogen synthesis, glucose 6-phosphate is converted to glucose 1-phosphate by phosphoglucomutase, a reversible reaction.
FIGURE 26.3 Scheme of glycogen synthesis and degradation. (S1) Glucose 6-phosphate is formed from glucose by hexokinase in most cells, and glucokinase in the liver. It is a metabolic branch point for the pathways of glycolysis, the pentose phosphate pathway, and glycogen synthesis. (S2) UDP-glucose (UDP-G) is synthesized from glucose 1-phosphate. UDP-glucose is the branch point for glycogen synthesis and other pathways that require the addition of carbohydrate units. (S3) Glycogen synthesis is catalyzed by glycogen synthase and the branching enzyme. (D1) Glycogen degradation is catalyzed by glycogen phosphorylase and a debrancher enzyme. (D2) Glucose 6-phosphatase in the liver (and, to a small extent, the kidney) generates free glucose from glucose 6-phosphate. ATP, adenosine triphosphate; Pi, inorganic phosphate; PPi, pyrophosphate; UTP, uridine triphosphate.
Glycogen is both formed from and degraded to glucose 1-phosphate, but the biosynthetic and degradative pathways are separate and involve different enzymes (see Fig. 26.3). The biosynthetic pathway is an energy-requiring pathway; high-energy phosphate from UTP is used to activate the glucosyl residues to UDP-glucose (Fig. 26.4). In the degradative pathway, the glycosidic bonds between the glucosyl residues in glycogen are simply cleaved by the addition of phosphate to produce glucose 1-phosphate (or water to produce free glucose), and UDP-glucose is not resynthesized. The existence of separate pathways for the formation and degradation of important compounds is a common theme in metabolism. Because the synthesis and degradation pathways use different enzymes, one can be activated while the other is inhibited.
FIGURE 26.4 Formation of UDP-glucose. The high-energy phosphate bond of UTP provides the energy for the formation of a high-energy bond in UDP-glucose. Pyrophosphate (PPi), released by the reaction, is cleaved to two inorganic phosphate (Pi).
A. Glycogen Synthesis
Glycogen synthesis requires the formation of α-1,4-glycosidic bonds to link glucosyl residues in long chains and the formation of an α-1,6-branch every 8 to 10 residues (Fig. 26.5). Most of glycogen synthesis occurs through the lengthening of the polysaccharide chains of a pre-existing glycogen molecule (a glycogen primer) in which the reducing end of the glycogen is attached to the protein glycogenin. To lengthen the glycogen chains, glucosyl residues are added from UDP-glucose to the nonreducing ends of the chain by glycogen synthase. The anomeric carbon of each glucosyl residue is attached in an α-1,4-bond to the hydroxyl on carbon 4 of the terminal glucosyl residue. When the chain reaches approximately 11 residues in length, a 6- to 8-residue piece is cleaved by amylo-4:6-transferase (also known as branching enzyme) and reattached to a glucosyl unit by an α-1,6-bond. Both chains continue to lengthen until they are long enough to produce two new branches. This process continues, producing highly branched molecules. Glycogen synthase, the enzyme that attaches the glucosyl residues in α-1,4-bonds, is the regulated step in the pathway. Branching of glycogen serves two major roles: increased sites for synthesis and degradation and enhancing the solubility of the molecule.
FIGURE 26.5 Glycogen synthesis. See text for details. UDP, uridine diphosphate.
The synthesis of new glycogen primer molecules also occurs. Glycogenin, the protein to which glycogen is attached, glycosylates itself (autoglycosylation) by attaching the glucosyl residue of UDP-glucose to the hydroxyl side chain of a serine residue in the protein. The protein then extends the carbohydrate chain (using UDP-glucose as the substrate) until the glucosyl chain is long enough to serve as a substrate for glycogen synthase.
B. Glycogen Degradation
Glycogen is degraded by two enzymes, glycogen phosphorylase and the debrancher enzyme (Fig. 26.6). Glycogen degradation is a phosphorolysis reaction (breaking of a bond using a phosphate ion as a nucleophile). Enzymes that catalyze phosphorolysis reactions are named phosphorylase. Because more than one type of phosphorylase exists, the substrate usually is included in the name of the enzyme, such as glycogen phosphorylase or purine nucleoside phosphorylase.
FIGURE 26.6 Glycogen degradation. See text for details. Pi, inorganic phosphate.
The enzyme glycogen phosphorylase starts at the nonreducing end of a chain and successively cleaves glucosyl residues by adding phosphate to the anomeric carbon of the terminal glycosidic bond, thereby releasing glucose 1-phosphate and producing a free 4′-hydroxyl group on the glucose residue now at the end of the glycogen chain. However, glycogen phosphorylase cannot act on the glycosidic bonds of the four glucosyl residues closest to a branch point because the branching chain sterically hinders a proper fit into the catalytic site of the enzyme. The debrancher enzyme, which catalyzes the removal of the four residues closest to the branch point, has two catalytic activities: it acts as a transferase and as an α-1,6-glucosidase. As a transferase, the debrancher first removes a unit containing three glucose residues and adds it to the end of a longer chain by an α-1,4-glycosidic bond. The one glucosyl residue remaining at the α-1,6-branch is hydrolyzed by the amylo-1,6-glucosidase activity of the debrancher, resulting in the release of free glucose. Thus, one glucose and approximately seven to nine glucose 1-phosphate residues are released for every branch point.
Some degradation of glycogen also occurs within lysosomes when glycogen particles become surrounded by membranes that then fuse with the lysosomal membranes. A lysosomal α-glucosidase hydrolyzes this glycogen to glucose.
IV. Disorders of Glycogen Metabolism
A series of inborn errors of metabolism, the glycogen storage diseases, result from deficiencies in the enzymes of glycogenolysis (Table 26.1). The diseases are labeled I through XI, and O. Several disorders have different subtypes, as indicated in the legend to Table 26.1. Glycogen phosphorylase, the key regulatory enzyme of glycogen degradation, is encoded by different genes in the muscle and liver (tissue-specific isozymes), and thus a person may have a defect in one and not the other.
TABLE 26.1 Glycogen Storage Diseases
TYPE | ENZYME AFFECTED (GENE) | PRIMARY ORGAN INVOLVED | MANIFESTATIONSa |
O | Glycogen synthase (GYS2) | Liver | Hypoglycemia, hyperketonemia, failure to thrive, early death |
Ib | Glucose 6-phosphatase (Von Gierke disease) (G6PC) | Liver | Enlarged liver and kidney, growth failure, severe fasting hypoglycemia, acidosis, lipemia, thrombocyte dysfunction |
II | Lysosomal α-glucosidase (Pompe disease): may see clinical symptoms in childhood, juvenile, or adult life stages, depending on the nature of the mutation (GAA) | All organs with lysosomes | Infantile form: early-onset progressive muscle hypotonia, cardiac failure, death before age 2 years. Juvenile form: later-onset myopathy with variable cardiac involvement. Adult form: limb-girdle muscular dystrophy-like features. Glycogen deposits accumulate in lysosomes |
III | Amylo-1,6-glucosidase (debrancher): form IIIa is the liver and muscle enzymes, form IIIb is a liver-specific form, and IIIc a muscle-specific form (AGL) | Liver, skeletal muscle, heart | Fasting hypoglycemia; hepatomegaly in infancy and some myopathic features. Glycogen deposits have short outer branches |
IV | Amylo-4,6-glucosidase (branching enzyme) (Andersen disease) (GBE1) | Liver | Hepatosplenomegaly; symptoms may arise from a hepatic reaction to the presence of a foreign body (glycogen with long outer branches). Usually fatal |
V | Muscle glycogen phosphorylase (McArdle disease) (expressed as either adult or infantile form) (PYGM) | Skeletal muscle | Exercise-induced muscular pain, cramps, and progressive weakness, sometimes with myoglobinuria |
VI | Liver glycogen phosphorylase (Her disease) (PYGL) | Liver | Hepatomegaly, mild hypoglycemia; good prognosis |
VII | Phosphofructokinase-I (Tarui syndrome) (PFKM) | Muscle, red blood cells | As in type V; in addition, enzymopathic hemolysis |
IXc | Phosphorylase kinase (PHKA2; PHKB; PHKG2; PHKA1) | Liver and muscle | Similar to type VI for liver-specific subunits; muscle fatigue for muscle-specific subunits |
Xd | Phosphoglycerate mutase (PGAM2) | Muscle | Similar to type V |
XII | Aldolas A (ALDOA) | Liver, red blood cells | Hepatosplenomegaly, hemolytic anemia |
aAll of these diseases except type O are characterized by increased glycogen deposits.
bGlucose 6-phosphatase is composed of several subunits that also transport glucose, glucose 6-phosphate, phosphate, and pyrophosphate across the endoplasmic reticulum membranes. Therefore, there are two major subtypes of this disease, corresponding to defects in the different subunits. Type Ia is a lack of glucose 6-phosphatase activity; type Ib is a lack of glucose 6-phosphate translocase activity (the SLC37A4 gene), which transfers glucose 6-phosphate from the cytosol to the endoplasmic reticulum, where glucose 6-phosphatase is located.
cGlycogen storage disease IX consists of a variety of subtypes. (IXa is the α-subunit of hepatic phosphorylase kinase; IXb is the common β-subunit for both liver and muscle phosphorylase kinase; IXc is the liver-specific γ-subunit, while IXd is the muscle-specific α-subunit.)
dGlycogen storage disease 11 is the muscle-specific subunit of lactate dehydrogenase (LDHA) with symptoms similar to GSD10; GSD13 is the muscle predominant form of enolase (ENO3), with symptoms similar to GSD10; and GSD15 is the muscle form of glycogenin (GYG1), with symptoms of muscle weakness and carbohydrate accumulation in the heart, leading to arrhythmias.
Data from Parker PH, Ballew M, Greene HL. Nutritional management of glycogen storage disease. Annu Rev Nutr. 1993;13:83–109; Shin YS. Glycogen storage disease: clinical, biochemical and molecular heterogeneity. Semin Pediatr Neurol. 2006;13:115–120; Ozen H. Glycogen storage diseases: new perspectives. World J Gastroenterol. 2007;13:2541-2553; The Genetics Home Reference database (ghr.nlm.nih.gov); search for the glycogen storage diseases.
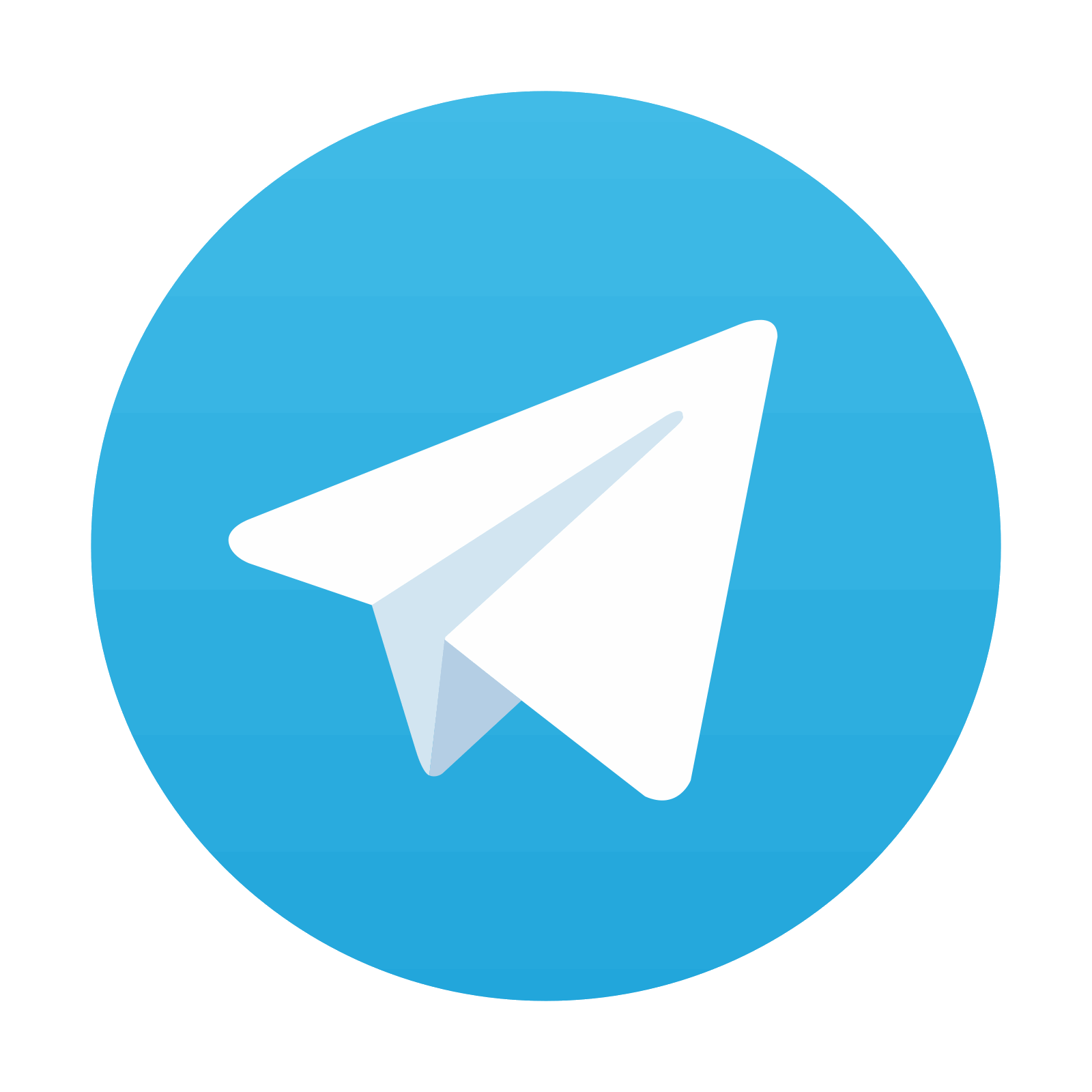
Stay updated, free articles. Join our Telegram channel
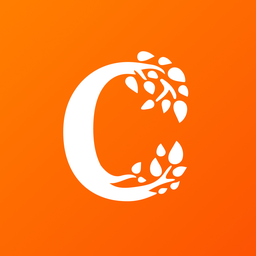
Full access? Get Clinical Tree
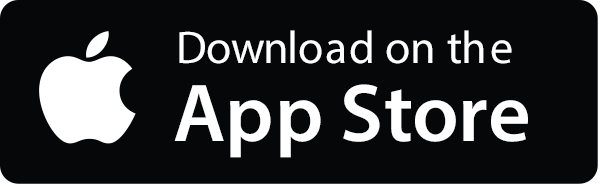
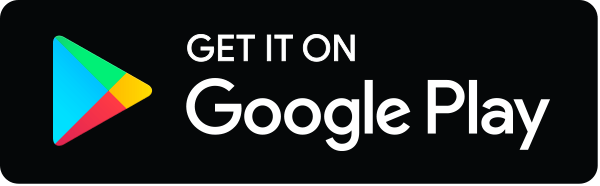