Enzymes are proteins that act as catalysts, which are compounds that increase the rate of chemical reactions (Fig. 8.1). Enzyme catalysts bind reactants (substrates), convert them to products, and release the products. Although enzymes may be modified during their participation in this reaction sequence, they return to their original form at the end. In addition to increasing the speed of reactions, enzymes provide a means for regulating the rate of metabolic pathways in the body. This chapter describes the properties of enzymes that allow them to function as catalysts. The next chapter explains the mechanisms of enzyme regulation.
FIGURE 8.1 Catalytic power of enzymes. Many enzymes increase the rate of a chemical reaction by a factor of 1011 or higher. To appreciate an increase in reaction rate by this order of magnitude, consider a room-sized box of golf balls that “react” by releasing energy and turning brown. The 12 ft × 12 ft × 8 ft box contains 380,000 golf balls. If the rate of the reaction in the absence of enzyme were 100 golf balls per year, the presence of 1 molecule of enzyme would turn the entire box of golf balls brown in 1 second (assuming a 1011 increase in reaction rate).
Enzyme-Binding Sites. An enzyme binds the substrates of the reaction and converts them to products. The substrates are bound to specific substrate-binding sites on the enzyme through interactions with the amino acid residues of the enzyme. The spatial geometry required for all the interactions between the substrate and the enzyme makes each enzyme selective for its substrates and ensures that only specific products are formed.
Active Catalytic Sites. The substrate-binding sites overlap in the active catalytic site of the enzyme, the region of the enzyme where the reaction occurs. Within the catalytic site, functional groups provided by coenzymes, tightly bound metals, and, of course, amino acid residues of the enzyme, participate in catalysis.
Activation Energy and the Transition State. The functional groups in the catalytic site of the enzyme activate the substrate and decrease the energy needed to form the high-energy intermediate stage of the reaction known as the transition-state complex. Some of the catalytic strategies employed by enzymes, such as general acid–base catalysis, formation of covalent intermediates, and stabilization of the transition state, are illustrated by chymotrypsin.
pH and Temperature Profiles. Enzymes have a functional pH range determined by the pKa of the functional groups in the active site and the interactions required for three-dimensional structure. Increases of temperature, which do not lead to protein denaturation, increase the reaction rate.
Mechanism-Based Inhibitors. The effectiveness of many drugs and toxins depends on their ability to inhibit an enzyme. The strongest inhibitors are covalent inhibitors, which are compounds that form covalent bonds with a reactive group in the enzyme active site, or transition-state analogs that mimic the transition-state complex.
Enzyme Names. Most enzyme names end in “-ase.” Enzymes usually have both a common name and a systematic classification that includes a name and an Enzyme Commission (EC) number.
THE WAITING ROOM 
A year after recovering from salicylate poisoning (see Chapter 4), Dennis V. was playing in his grandfather’s basement. Dennis V. drank an unknown amount of the insecticide malathion, which is sometimes used for killing fruit flies and other insects (Fig. 8.2). Sometime later, when he was not feeling well, he told his grandfather what he had done. His grandfather retrieved the bottle and rushed Dennis V. to the emergency room of the local hospital. On the way, Dennis V. vomited repeatedly and complained of abdominal cramps. At the hospital, he began salivating and had an uncontrollable defecation.
FIGURE 8.2 Organophosphorus compounds. Malathion and parathion are organophosphorus insecticides. Nausea, coma, convulsions, respiratory failure, and death have resulted from the use of parathion by farmers who have gotten it on their skin. Malathion is similar in structure to parathion but is not nearly as toxic. The nerve gas sarin, another organophosphorus compound, was used in a terrorist attack in a subway in Japan.
In the emergency room, physicians passed a nasogastric tube for stomach lavage, started intravenous fluids, and recorded vital signs. Dennis V.’s pulse rate was 48 beats/minute (slow), and his blood pressure was 75/42 mm Hg (low). The physicians noted involuntary twitching of the muscles in his extremities.
Lotta T. was diagnosed with acute gouty arthritis involving her right great toe (see Chapter 5). The presence of insoluble urate crystals within the joint space confirmed the diagnosis. Several weeks after her acute gout attack subsided, she was started on allopurinol therapy in an oral dose of 150 mg twice per day, while continuing a daily dose of colchicine. Allopurinol therapy is effective because the drug inhibits the activity of a specific enzyme.
Al M., a 44-year-old male who has suffered from alcohol use disorder for the past 5 years, has a markedly diminished appetite for food. One weekend, he became unusually irritable and confused after drinking two 750 ml bottles of vodka and eating very little. His landlady convinced him to visit his doctor. Physical examination indicated a heart rate of 104 beats/minute. His blood pressure was slightly low, and he was in early congestive heart failure. He was poorly oriented to time, place, and person.
I. The Enzyme-Catalyzed Reaction
Enzymes, in general, provide speed, specificity, and regulatory control to reactions in the body. Enzymes are usually proteins that act as catalysts, which are compounds that increase the rate of chemical reactions. Enzyme-catalyzed reactions have three basic steps:
- Binding of substrate (a reactant): E + S ↔ ES
- Conversion of bound substrate to bound product: ES ↔ EP
- Release of product: EP ↔ E + P
An enzyme binds the substrates of the reaction it catalyzes and brings them together at the right orientation to react. The enzyme then participates in the making and breaking of bonds required for product formation, releases the products, and returns to its original state once the reaction is completed.
Enzymes do not invent new reactions; they simply make reactions occur faster. The catalytic power of an enzyme (the rate of the catalyzed reaction divided by the rate of the uncatalyzed reaction) is usually in the range of 106 to 1014. Without the catalytic power of enzymes, reactions such as those involved in nerve conduction, heart contraction, and digestion of food would occur too slowly for life to exist.
Each enzyme usually catalyzes a specific biochemical reaction. The ability of an enzyme to select just one substrate and distinguish this substrate from a group of very similar compounds is referred to as specificity (Fig. 8.3). The enzyme converts this substrate to just one product. The specificity as well as the speed of enzyme-catalyzed reactions result from the unique sequence of specific amino acids that form the three-dimensional structure of the enzyme.
FIGURE 8.3 Reaction catalyzed by glucokinase, an example of enzyme reaction specificity. Glucokinase catalyzes the transfer of a phosphate (P) from adenosine triphosphate (ATP) to carbon 6 of glucose. It cannot rapidly transfer a phosphate from other nucleotides to glucose, or from ATP to closely related sugars such as galactose, or from ATP to any other carbon on glucose. The only products formed are glucose 6-phosphate and adenosine diphosphate (ADP).
A. The Active Site
To catalyze a chemical reaction, the enzyme forms an enzyme–substrate complex in its active catalytic site (Fig. 8.4). The active site is usually a cleft or crevice in the enzyme formed by one or more regions of the polypeptide chain. Within the active site, cofactors and functional groups from the polypeptide chain participate in transforming the bound substrate molecules into products.
FIGURE 8.4 Reaction in the enzyme active catalytic site. A. The enzyme contains an active catalytic site, shown in dark red, with a region or domain where the substrate binds. The active site also may contain cofactors—nonprotein components that assist in catalysis. B. The substrate forms bonds with amino acid residues in the substrate-binding site. Substrate binding induces a conformational change in the active site. C. Functional groups of amino acid residues and cofactors in the active site participate in forming the transition-state complex, which is stabilized by additional noncovalent bonds with the enzyme, shown in red. D: Because the products of the reaction dissociate, the enzyme returns to its original conformation.
Initially, the substrate molecules bind to their substrate-binding sites, also called the substrate-recognition sites (see Fig. 8.4B). The three-dimensional arrangement of binding sites in a crevice of the enzyme allows the reacting portions of the substrates to approach each other from the appropriate angles. The proximity of the bound substrate molecules and their precise orientation toward each other contribute to the catalytic power of the enzyme.
The active site also contains functional groups that participate directly in the reaction (see Fig. 8.4C). The functional groups are donated by the polypeptide chain, or by bound cofactors (metals or complex organic molecules called coenzymes). As the substrate binds, it induces conformational changes in the enzyme that promote further interactions between the substrate molecules and the enzyme functional groups. (For example, a coenzyme might form a covalent intermediate with the substrate, or an amino acid side chain might abstract a proton from the reacting substrate.) The activated substrates and the enzyme form a transition-state complex, an unstable high-energy complex with a strained electronic configuration that is intermediate between substrate and product. Additional bonds with the enzyme stabilize the transition-state complex and decrease the energy required for its formation.
The transition-state complex decomposes to products, which dissociate from the enzyme (see Fig. 8.4D). The enzyme generally returns to its original form. The free enzyme then binds another set of substrates and repeats the process.
B. Substrate-Binding Sites
Enzyme specificity (the enzyme’s ability to react with just one substrate) results from the three-dimensional arrangement of specific amino acid residues in the enzyme that form binding sites for the substrates and activate the substrates during the course of the reaction. The “lock-and-key” and the “induced-fit” models for substrate binding describe two aspects of the binding interaction between the enzyme and substrate.
1. Lock-and-Key Model for Substrate Binding
The substrate-binding site contains amino acid residues arranged in a complementary three-dimensional surface that “recognizes” the substrate and binds it through multiple hydrophobic interactions, electrostatic interactions, or hydrogen bonds. The amino acid residues that bind the substrate can come from very different parts of the linear amino acid sequence of the enzyme, as seen in glucokinase. The binding of compounds with a structure that differs from the substrate even to a small degree may be prevented by steric hindrance and charge repulsion. In the lock-and-key model, the complementarity between the substrate and its binding site is compared to that of a key fitting into a rigid lock.
2. Induced-Fit Model for Substrate Binding
Complementarity between the substrate and the binding site is only part of the picture. As the substrate binds, enzymes undergo a conformational change (“induced fit”) that repositions the side chains of the amino acids in the active site and increases the number of binding interactions (see Fig. 8.4). The induced-fit model for substrate binding recognizes that the substrate-binding site is not a rigid “lock” but rather a dynamic surface created by the flexible overall three-dimensional structure of the enzyme.
The function of the conformational change induced by substrate binding, the induced fit, is usually to reposition functional groups in the active site in a way that promotes the reaction, improves the binding site of a cosubstrate, or activates an adjacent subunit through cooperativity. For example, consider the large conformational changes that occur in the actin fold of glucokinase when glucose binds. First, the substrate glucose binds in a manner resembling a lock and key (Fig. 8.5). As glucose binds, the induced fit results. The induced fit involves changes in the conformation of the whole enzyme that close the cleft of the fold, thereby improving the binding site for adenosine triphosphate (ATP), and excluding water (which might interfere with the reaction) from the active site (Fig. 8.6). Thus, the multiple interactions between the substrate and the enzyme in the catalytic site serve both for substrate recognition and for initiating the next stage of the reaction, formation of the transition-state complex.
FIGURE 8.5 Glucose-binding site in glucokinase. A. Glucose, shown in red, is held in its binding site by multiple hydrogen bonds between each hydroxyl group and polar amino acids from different regions of the enzyme amino acid sequence in the actin fold (see Chapter 7). The position of the amino acid residue in the linear sequence is given by its number. The multiple interactions enable glucose to induce large conformational changes in the enzyme (induced fit). (Modified from Pilkis SJ, Weber IT, Harrison RW, et al. Glucokinase: structural analysis of a protein involved in susceptibility to diabetes. J Biol Chem. 1994;269(35):21925–21928. https://creativecommons.org/licenses/by/4.0/) B. Enzyme specificity is illustrated by the comparison of galactose and glucose. Galactose differs from glucose only in the position of the –OH group shown in red. However, it is not phosphorylated at a significant rate by the enzyme. Cells therefore require a separate galactokinase for the metabolism of galactose.
FIGURE 8.6 Conformational change resulting from the binding of glucose to hexokinase. (The figure is actually yeast hexokinase, which is more similar to human glucokinase than it is to the other human hexokinase isozymes.)The shaded and unshaded areas show the two domains (four subdomains) that form the actin fold with its adenosine triphosphate (ATP)-binding cleft. A. Free enzyme. B. With glucose bound, the cleft closes, forming the ATP-binding site. The closure of the cleft when glucose binds to hexokinase (or human glucokinase) is one of the largest “induced fits” known. (Reprinted from Bennett WS Jr, Steitz TA. Structure of a complex between yeast hexokinase A and glucose: II. Detailed comparisons of conformation and active site configuration with the native hexokinase B monomer and dimer. J Mol Biol. 1980;140(2):211–230. © 1980 Elsevier. With permission.)
C. The Transition-State Complex
In order for a reaction to occur, the substrates undergoing the reaction need to be activated. If the energy levels of a substrate are plotted as the substrate is progressively converted to product, the curve will show a maximum energy level that is higher than that of either the substrate or the product (Fig. 8.7). This high energy level occurs at the transition state. For some enzyme-catalyzed reactions, the transition state is a condition in which bonds in the substrate are maximally strained. For other enzyme-catalyzed reactions, the electronic configuration of the substrate becomes very strained and unstable as it enters the transition state. The highest energy level corresponds to the most unstable substrate configuration, and the condition in which the changing substrate molecule is most tightly bound to participating functional groups in the enzyme. The difference in energy between the substrate and the transition-state complex is called the activation energy.
FIGURE 8.7 Energy diagram showing the energy levels of the substrates as they progress toward products in the absence of enzyme. The substrates must pass through the high-energy transition state during the reaction. Although a favorable loss of energy occurs during the reaction, the rate of the reaction is slowed by the energy barrier to forming the transition state. The energy barrier is referred to as the activation energy.
According to transition-state theory, the overall rate of the reaction is determined by the number of molecules acquiring the activation energy necessary to form the transition-state complex. Enzymes increase the rate of the reaction by decreasing this activation energy. They use various catalytic strategies, such as electronic stabilization of the transition-state complex or acid–base catalysis, to obtain this decrease.
Once the transition-state complex is formed, it can collapse back to substrates or decompose to form products. The enzyme does not change the initial energy level of the substrates or the final energy level of the products.
Because the transition-state complex binds more tightly to the enzyme than does the substrate, compounds that resemble its electronic and three-dimensional surface (transition-state analogs) are more potent inhibitors of an enzyme than are substrate analogs. Consequently, a drug developed as a transition-state analog would be highly specific for the enzyme it is designed to inhibit. However, transition-state analogs are highly unstable when they are not bound to the enzyme and would have great difficulty making it from the digestive tract or injection site to the site of action. Some of the approaches in drug design that are being used to deal with the instability problem include designing drugs that are almost transition-state analogs but have a stable modification; designing a prodrug that is converted to a transition-state analog at the site of action; and using the transition-state analog to design a complementary antibody.
If the structure of a transition state can be modeled, it can be used as an antigen for the production of abzymes (catalytic antibodies). These antibodies have an arrangement of amino acid side chains in their variable regions that is similar to the active site of the enzyme in the transition state. Consequently, they can act as artificial enzymes. For example, abzymes have been developed against analogs of the transition-state complex of cocaine esterase, the enzyme that degrades cocaine in the body. These abzymes have esterase activity, and monthly injections of the abzyme drug can be used to rapidly destroy cocaine in the blood, thereby decreasing the dependence of addicted individuals. (See Chapter 7 for antibody structure.)
II. Strategies for Catalysis
There are five major strategies used by enzymes to enable catalysis: general acid–base catalysis, covalent catalysis, metal-ion catalysis, catalysis by approximation, and cofactor catalysis. Some enzymes will use a combination of these strategies.
A. General Acid–Base Catalysis
In general acid–base catalysis, a functional group on the protein either donates a proton (acid catalysis) or accepts a proton (general base catalysis) during the course of the reaction. An example of general acid–base catalysis is seen in the mechanism of chymotrypsin (see the online Catalytic Mechanism of Chymotrypsin material). During the course of this reaction, histidine 57 acts as a general base catalyst and accepts a proton from serine 195, activating the serine to act as a nucleophile. Later on in the reaction sequence, the protonated histidine 57 acts as a general acid catalyst and donates a proton to a product leaving the reaction.
B. Covalent Catalysis
In covalent catalysis, the substrate is covalently linked during the course of the reaction to an amino acid side chain at the active site of the enzyme. Chymotrypsin (a protease, an enzyme which breaks peptide bonds) also exhibits covalent catalysis. Once histidine 57 activates serine 195 by removing its proton from the hydroxyl group on the side chain, the negatively charged oxyanion attacks the carbonyl group of the peptide bond to be cleaved by the enzyme, forming a covalent bond and tetrahedral intermediate. The substrate stays covalently linked to the enzyme through the course of the reaction.
C. Metal-Ion Catalysis
Many enzymes contain required metal ions to allow catalysis to occur. In the case of carbonic anhydrase, an enzyme-bound zinc at the active site binds and orients water appropriately so it can participate in the reaction. In the absence of the active-site zinc, the reaction occurs very slowly, if at all.
D. Catalysis by Approximation
Catalysis by approximation refers to the enzyme forcing (through the formation of hydrogen bonds and ionic interactions between the enzyme and substrate) substrates to bind in a manner that places reactive groups in the appropriate orientation so a reaction can take place. Nucleoside monophosphate kinases use this type of mechanism to transfer a phosphate from a nucleoside triphosphate to a nucleoside monophosphate, producing two nucleoside diphosphates.
E. Cofactor Catalysis
In cofactor catalysis, a required cofactor for an enzyme usually forms a covalent bond with the substrate during the course of the reaction. Enzymes involved in amino acid metabolism use pyridoxal phosphate (derived from vitamin B6) to form a covalent bond during the course of the reaction.
III. Functional Groups in Catalysis
The catalytic strategies as described in the previous section to increase the reaction rate are common to many enzymes. A variety of functional groups are employed by different enzymes to carry out these catalytic strategies. Some enzymes, such as chymotrypsin, rely on amino acid residues within the active site. Other enzymes increase their repertoire by employing cofactors (nonprotein compounds that participate in the catalytic process) to provide a functional group with the right size, shape, and properties. They are generally divided into three categories: coenzymes (such as pyridoxal phosphate), metal ions (e.g., Fe2+, Mg2+, or Zn2+), and metallocoenzymes (similar to the Fe2+-heme in hemoglobin, see Chapter 7).
A. Functional Groups on Amino Acid Side Chains
Almost all of the polar amino acids participate directly in catalysis in one or more enzymes (Table 8.1). Serine, cysteine, lysine, and histidine can participate in covalent catalysis. Histidine, because it has a pKa that can donate and accept a proton at neutral pH, often participates in acid–base catalysis. Most of the polar amino acid side chains are nucleophilic and participate in nucleophilic catalysis by stabilizing more positively charged groups that develop during the reaction.
TABLE 8.1 Some Functional Groups in the Active Site
FUNCTION OF AMINO ACID | ENZYME EXAMPLE |
Covalent intermediates | |
Cysteine–SH | Glyceraldehyde 3–phosphate dehydrogenase |
Serine–OH | Acetylcholinesterase, chymotrypsin |
Lysine–NH2 | Aldolase |
Histidine–NH | Phosphoglucomutase |
Acid–base catalysis | |
Histidine–NH | Chymotrypsin |
Aspartate–COOH | Pepsin |
Stabilization of anion formed during the reaction | |
Peptide backbone–NH | Chymotrypsin |
Arginine–NH | Carboxypeptidase A |
Serine–OH | Alcohol dehydrogenase |
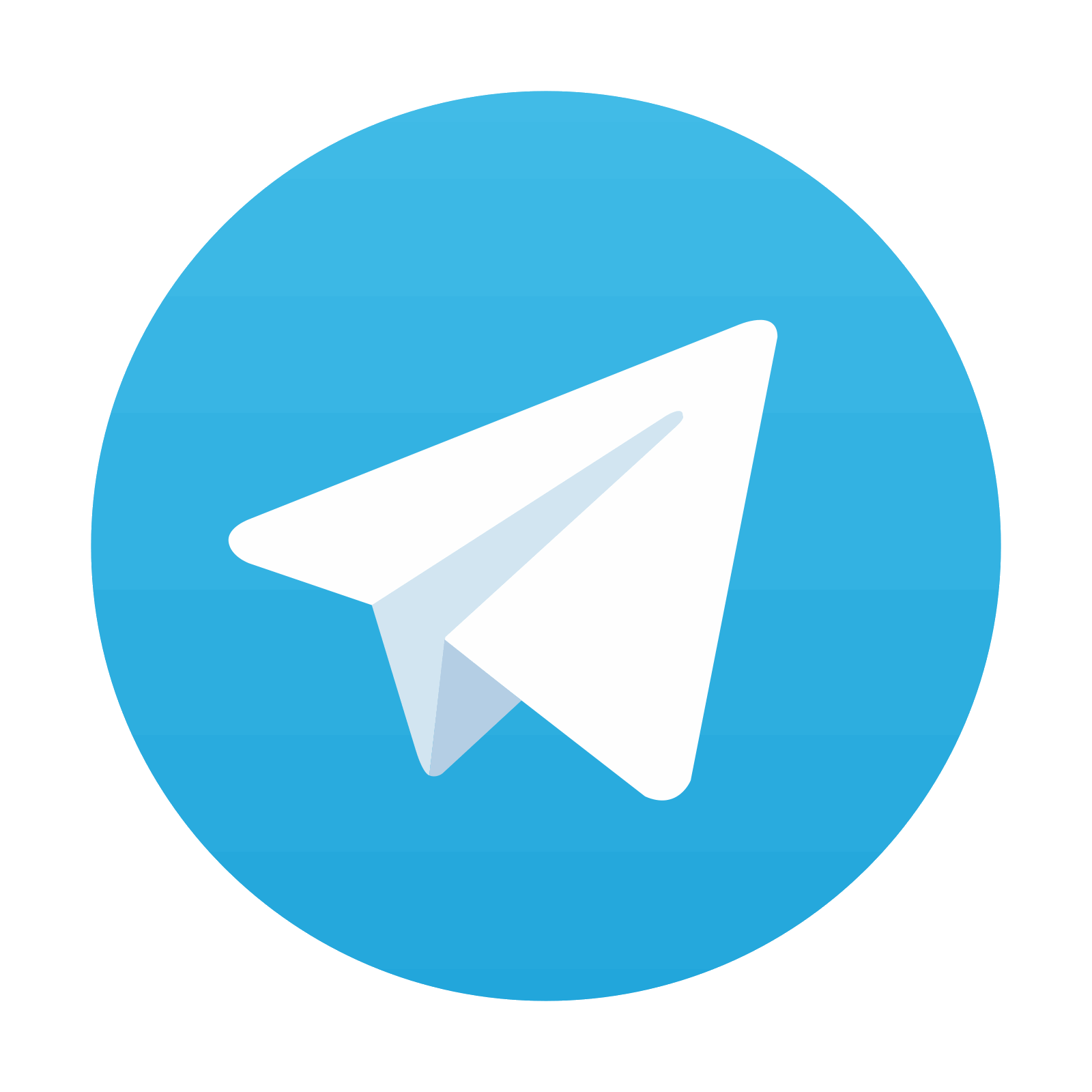
Stay updated, free articles. Join our Telegram channel
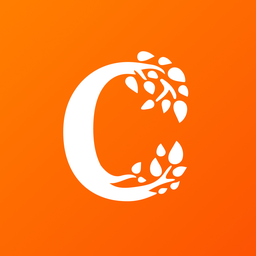
Full access? Get Clinical Tree
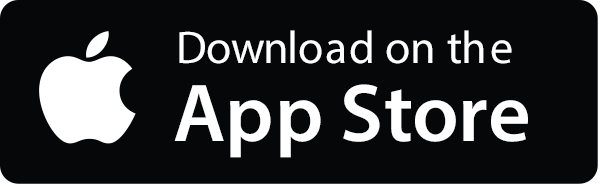
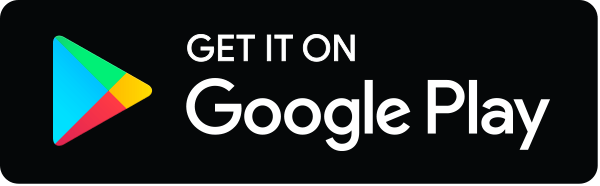